Figure 1: Triple protostar system L1448 IRS3B, showing a central binary pair of protostars (IRS3B-a & IRS3B-b, with a combined mass of ∼1 M☉), orbited by a less-massive but much-brighter companion protostar (IRS3B-c, with a mass of ∼0.085 M☉) in a circumbinary orbit.
An alternative ideology, presented here, suggests that the luminosity difference results from age difference, in a stellar system that turned itself inside out by a flip-flop mechanism, designated, symmetrical flip-flop fragmentation (symmetrical FFF). The companion originally formed as the central protostar, followed by a dual disk instability in two arms of a (spiral) density wave of a stellar-mass accretion disk. The resulting twin disk-instability objects were much-more massive than the diminutive protostellar core, creating a dynamically-unstable system. Equipartition of kinetic energy during orbital interplay gradually flip-flopped the former stellar core into a circumbinary orbit around the much-more-massive but younger binary pair, forming a hierarchical trinary protostar system.
Image Credit: Bill Saxton, ALMA (ESO/NAOJ/NRAO), NRAO/AUI/NSF – Publication: John Tobin (Univ. Oklahoma/Leiden) et al.
Abstract:
This alternative conceptual ideology suggests three novel mechanisms for the formation of gravitationally-bound celestial objects:
– Flip-flop fragmentation (FFF)
– Trifurcation
– Hybrid accretion
– Flip-flop fragmentation (FFF)—a suggested flip-flop mechanism for forming pairs or trios of objects with mass disparity:
¶ FFF suggests that prestellar/very-early protostellar accretion disks are much-more massive than their diminutive prestellar cores, such that the accretion disk dominates the system. Inertial dominance promotes disk instability, forming either a solitary disk-instability (d-i) object or a twin pair of d-i objects, where the d-i objects are necessarily more massive than their diminutive core. Spiral density waves may promote disk instability, with an asymmetrical (m = 1 mode) density wave, condensing a solitary d-i object, or a symmetrical (m = 2 mode) density wave, condensing a twin pair of d-i objects. Inherent in asymmetrical FFF is an inertial flip-flop, where the diminutive prestellar/protostellar core is injected into a satellite orbit around the more-massive nascent d-i object. In symmetrical FFF, the flip-flop occurs gradually by means of orbital interplay between the diminutive core and the more-massive twin d-i objects.
¶ FFF suggests that most gas-giant planets are former stellar cores that predate their stellar hosts, with giant-planet multiplicity generally the result of multiple, sequential asymmetrical FFF occurrences.
– Asymmetrical FFF—Asymmetrical FFF forms a solitary d-i object that inertially displaces the diminutive former stellar core into planetary satellite orbit. Asymmetrical FFF forms satellite objects in the size range of mini-Neptunes to low-mass brown dwarfs.
– Symmetrical FFF—Symmetrical FFF forms a twin pair of d-i objects around a diminutive prestellar/protostellar core, creating a dynamically unstable system. Subsequent orbital interplay ‘evaporates’ the diminutive, former stellar core into a circumbinary orbit around the twin binary pair, creating a stable hierarchical system. While asymmetrical FFF predominantly forms planetary-mass planets, symmetrical FFF predominantly forms brown dwarf and red dwarf companions to binary stellar pairs. The Alpha Centauri system presumably formed by symmetrical FFF, with Proxima Centauri as the original stellar core, which was ‘evaporated’ into a circumbinary orbit around the much-more-massive twin binary pair of d-i objects (Alpha Centauri A&B). Many twin asteroids and Kuiper belt objects presumably also formed by symmetrical FFF.
– Trifurcation—a suggested centrifugal fragmentation mechanism for forming twin-binary pairs, such as Jupiter-Saturn, Uranus-Neptune, and Venus-Earth, like Russian nesting dolls:
¶ Trifurcation may be a possible secondary effect of symmetrical FFF. During the orbital interplay phase of symmetrical FFF, orbital close encounters between a diminutive protostellar core and it’s more-massive twin d-i objects evolve toward equipartition of kinetic energy, which is a thermodynamic effect that transfers orbital kinetic energy and angular momentum from the more massive d-i objects to the less-massive protostellar core. And if equipartition of kinetic energy is partially rotational, it may cause spin up to the point of centrifugal fragmentation. Spin up initially causes flattening into an oblate sphere, followed by distortion into a tri-axial Jacobi ellipsoid, and then into a bar-mode instability. The failure mode of a bar-mode instability is suggested here to result in centrifugal fragmentation into 3 components, hence trifurcation. During trifurcation, the bar-mode arms gravitationally pinch off into independent Roche spheres, forming a binary pair of gravitationally-bound spheres in orbit around the diminutive ‘residual core’ of material remaining at the center of rotation.
¶ This newly trifurcated system, composed of a twin binary pair in orbit around its diminutive residual core is a Mini-Me version of the original symmetrical FFF system, and like the original system, the newly-trifurcated system is also dynamically unstable. Thus, first-generation trifurcation promotes second-generation trifurcation, and etc., potentially forming multiple-generations of twin binary pairs of objects in diminishing sizes like Russian nesting dolls. Trifurcation is the suggested origin of the four sets of twin-binary pairs in our solar system, namely, former ‘binary-Companion’, Jupiter-Saturn, Uranus-Neptune, and Venus-Earth, with the planet Mercury as the residual core of the 4th generation trifurcation. (Mars was formed otherwise.) Alpha Centauri system presumably also formed by symmetrical FFF, but did not result in trifurcation.
¶ Binary asteroids and Kuiper belt objects presumably also formed by symmetrical FFF, and some may have undergone trifurcation as well, namely the Pluto system, with Pluto and Charon as the twin d-i objects, where Nix-Hydra are 1st-generation trifurcation twins and Styx-Kerberos are 2nd-generation twins.
– Hybrid accretion—a mechanism for forming super-Earths and giant-planet moons:
¶ Hybrid accretion is a suggested hybrid planet formation mechanism (Thayne Curie 2005), combining planetesimal formation by streaming instability, followed by hierarchical accretion into planets capable of clearing their orbits.
¶ Planetesimals form by streaming instability at the inner edge of a protoplanetary disk, possibly against the star’s magnetic corotation radius. When hierarchical accretion reaches the nominal mass of a super-Earth, the newly-formed planet is able to clear its orbit, promoting the formation of second-generation planetesimals by streaming instability against the outer resonances of the newly-formed super-Earth. In this manner, a cascade of super-Earths may form from the inside out. Cascades of hybrid-accretion moons may form around gas-giant and ice-giant planets.
A brief history of the solar system:
1) Symmetrical FFF―forming binary-Sun (twin d-i objects) + Brown Dwarf* (protostellar core)
2) 1st-generation trifurcation―forming binary-Companion + SUPER-Jupiter* (residual core)
3) 2nd-gen. trifurcation―forming Jupiter-Saturn + SUPER-Neptune* (residual core)
4) 3rd-gen. trifurcation―forming Uranus-Neptune + SUPER-Earth* (residual core)
5) 4th-gen. trifurcation―forming Venus-Earth + Mercury (residual core)
¶ A diminutive protostar with a much-more-massive accretion disk underwent symmetrical FFF, forming a twin pair of d-i objects > 4,567 Ma. The brown-dwarf-mass protostellar core underwent four generations of trifurcation as a secondary effect of symmetrical FFF. And the resulting high-angular-momentum ‘trifurcation debris disk’ formed hot classical Kuiper belt objects (KBOs) against Neptune’s outer 2:3 resonance.
¶ The descendants of Brown Dwarf and the trifurcation debris disk all lay on the 3-oxygen-isotope ‘Brown Dwarf fractionation line’, which we know as the ‘terrestrial fractionation line’. Additionally, assuming Brown Dwarf had internally differentiated, the hot-classical KBOs formed from the trifurcation debris disk are siderophile depleted and lay on the terrestrial fractionation line, comparable to the lithophile continental crust on Earth.
¶ Following 4th-generation trifurcation, binary-Companion presumably orbited binary-Sun at about 15 AU, with the 2nd, 3rd, and 4th-gen. trifurcation components still within binary-Companion’s gravity well. Binary-binary resonances unwound the trifurcation generations, presumably by eccentricity pumping, causing Uranus-Neptune to be captured by binary-Sun by way of binary-Companion’s outer L2 Lagrangian point. Jupiter-Saturn, with Venus-Earth-Mercury in tow, were captured by binary-Sun via binary-Companion’s inner L1 Lagrangian point. Then Venus-Earth-Mercury were captured from Jupiter-Saturn via the L1 Lagrangian point. Finally, additional eccentricity pumping caused twin-binary trifurcation pairs to separate. Mars is unaccounted for in this tally, with its size and composition possibly indicating formation by hybrid accretion around former Brown Dwarf, prior to symmetrical FFF.
¶ The twin-binary d-i objects of symmetrical FFF spiraled-in to become our former binary-Sun and merged at 4,567 Ma in a luminous red nova that created a low-angular-momentum ‘solar-merger debris disk’. The solar-merger debris disk, with stellar-merger short-lived radionuclides (SLRs), formed asteroids by streaming instability, likely against the Sun’s merger-enlarged magnetic corotation radius. Slightly later, after the SLRs died away, chondrites formed in situ by streaming instability against Jupiter’s strongest inner resonances.
¶ Secular perturbation caused the super-Jupiter-mass components of former binary-Companion (orbiting the Sun between Saturn and Uranus) to spiral in, translating their binary kinetic energy to the heliocentric orbit, progressively increasing the heliocentric period and eccentricity of former binary-Companion. The outer 1:4 mean-motion resonance with binary-Companion swept through the Plutinos followed by the cubewanos, perturbing KBOs which caused a bimodal late heavy bombardment of the inner solar system around 4 Ga. And the progressively-increasing eccentricity of former binary-Companion overran Uranus’ orbit, causing its 98º obliquity. At 650 Ma, the binary components merged in an asymmetrical merger explosion that gave the newly-merged Companion escape velocity from the Sun and fogged the solar system, causing the Marinoan glaciation on Earth of the Cryogenian period. The high-angular-momentum ‘Companion-merger debris disk’ formed the young (650 Ma) cold classical Kuiper belt objects in situ against Neptune’s outer 2:3 resonance.
* Note, unorthodox capitalization indicates unorthodox definitions. ‘SUPER-Jupiter’, ‘SUPER-Neptune’ and ‘SUPER-Earth’ are the names for the former residual cores formed in the first three trifurcation generations, and ‘Brown Dwarf’ is the name of the original prestellar core of the solar system.
………………..
Introduction:
¶ This alternative solar system formation hypothesis is an attempt at unifying formerly disparate solar system phenomena into a deterministic clockwork hypothesis that is more fertile than the standard model, with greater explanatory and predictive power and greater falsifiability; however, this alternative approach requires new speculative mechanisms and completely lacks any mathematical foundation. If fertile hypotheses are easy to formulate, then this particular hypothesis deserves no notice, but if fertile hypotheses are fiendishly difficult to devise unless accurate, then this effort may warrant further attention. Lest its clockwork nature be held against it, recall that both Grand Tack and the Nice model are also clockwork models.
¶ The trifurcation (Russian nesting doll) concept derived from an attempt to explain the 3 sets of twin planets in our highly-unusual solar system in a deterministic rather than ad hoc manner, by which mechanism planets are always formed in pairs, and in a graduated size-and-density progression between trifurcation generations. Two guiding principles of this philosophy are that primary mechanisms (determinism) are always superior to ad hoc secondary mechanisms (‘statisticalism’), and that catastrophism is generally preferable to gradualism.
Star formation stages:
¶ 1) Starless core: May be a transient phase or may progress to Jeans instability
¶ 2) Prestellar core: The (Jeans instability) collapse phase is designated ‘prestellar core’. Gravitational collapse is divided into two phases, separated by a brief, transitory first hydrostatic core (FHSC) that occurs when molecular hydrogen endothermically dissociates into atomic hydrogen around 2000 K. The FHSC is followed by ‘second collapse’, which ends with the formation of the second hydrostatic core (SHSC).
¶ 3) Protostar (Class 0, I, II, III): Begins with the formation of the second hydrostatic core
¶ 4) Pre-main-sequence star: A T Tauri, FU Orionis, or larger (unnamed) pre-main-sequence star powered by gravitational contraction
¶ 5) Main-sequence star: Powered by hydrogen fusion
¶ “Starless cores are possibly transient concentrations of molecular gas and dust without embedded young stellar objects (YSOs), typically observed in tracers such as C18O (e.g. Onishi et al. 1998), NH3 (e.g. Jijina, Myers, & Adams 1999), or dust extinction (e.g. Alves et al. 2007), and which do not show evidence of infall. Prestellar cores are also starless (M⋆ = 0) but represent a somewhat denser and more centrally-concentrated population of cores which are self-gravitating, hence unlikely to be transient.” (André et al. 2008)
¶ In Jeans instability, the cloud collapses at an approximately free-fall rate nearly isothermally at about 10 K until the center become optically thick at ~1013 g/cm3 after 105 yr (Larson 1969), at which point when the temperature begins to rise, forming a ‘first core’ or first hydrostatic core (FHSC). Supersonically infalling gas in the envelope is decelerated and thermalized at the surface of the first core (Masunaga et al. 1998).
¶ When the temperature reaches about 2000 K, the hydrogen begins to dissociate endothermically, forming a ‘second core’, the birth of a protostar. The protostar grows in mass by accreting the infalling material from the circumstellar envelope, while the protostar keeps its radius at ~4 R☉ during the main accretion phase. (Masunaga et al. 1998)
¶ “The size of the first core was found to vary somewhat in the different simulations (more unstable clouds form smaller first cores) while the size, mass, and temperature of the second cores are independent of initial cloud mass, size, and temperature.
Conclusions. Our simulations support the idea of a standard (universal) initial second core size of ~3×10-3 AU and mass ~1.4×10-3 M☉.”
(Vaytet et al. 2013)
FHSC:
¶ The development of a bar mode and spiral structure is expected for rapidly rotating polytropic-like structures (e.g. Durisen et al. 1986). Such instabilities occur when the ratio of the rotational energy to the magnitude of the gravitational potential energy of the first core exceeds β = 0.274. Bate was also the first to point out that because a rapidly rotating first core develops into a disc before the stellar core forms, the disc forms before the star. Rather than hydrostatic cores, such structures are better described as ‘pre-stellar discs’.
¶ Without rotation (β = 0), the first core has an initial mass of ≈5 MJ and a radius of ≈5 au (in agreement with Larson 1969). However, with higher initial rotation rates of the molecular cloud core, the first cores become progressively more oblate. For example, with β = 0.005 using radiation hydrodynamics, before the onset of dynamical instability, the first core has a radius of ≈20 au and a major-to-minor-axis ratio of ≈4:1. With β = 0.01, the first core has a radius of ≈30 au and a major-to-minor-axis ratio of ≈6:1. Thus, for the higher rotation rates, the first core is actually a pre-stellar disc, without a central object. As pointed out by Bate (1998), Machida et al. (2010) and Bate (2010), the disc actually forms before the star. For the very highest rotation rates (β = 0.04), the first core actually takes the form of a torus or ring in which the central density is lower than the maximum density.
¶ In each of the β = 0.001–0.005 cases, the first core begins as an axisymmetric flattened pre-stellar disc, but after several rotations, it develops a bar mode. The ends of the bar subsequently lag behind and the bar winds up to produce a spiral structure. Spiral structure removes angular momentum from the inner parts of the first core via gravitational torques (Bate 1998).
(Matthew R. Bate, 2011)
¶ “Class 0 objects are the youngest accreting protostars observed right after point mass formation, when most of the mass of the system is still in the surrounding dense core/envelope (Andre et al. 2000).”
(Chen et al. 2012)
¶ “Enoch et al. (2009a) discovered a massive circumstellar disk of ∼1 M☉ comparable to a central protostar around a Class 0 object, indicating that (1) the disk already exists in the main accretion phase and (2) the disk mass is significantly larger than the theoretical
prediction.” (Machida et al. 2011)
Protoplanetary disks have their highest masses at early times:
¶ “We find that the compact (< 100) dust emission is lower for Class I sources (median dust mass 96 M⊕) relative to Class 0 (248 M⊕), but several times higher than in Class II (5-15 M⊕). If this compact dust emission is tracing primarily the embedded disk, as is likely for many sources, this result provides evidence for decreasing disk masses with protostellar evolution, with sufficient mass for forming giant planet cores primarily at early times.” (Tychoniec et al. 2018)
¶ “The compact components around the Class 0 protostars could be the precursors to these Keplerian disks. However, it is unlikely that such massive rotationally supported disks could be stably supported given the expected low stellar mass for the Class 0 protostars: they should be prone to fragmentation”. (Li et al. 2014)
¶ The discovery that accretion disks are born massive and rapidly diminish with age is counterintuitive and may be problematic for the accretion theory formation of gas-giant planets, where the precipitous falloff in mass is fighting against the logarithmically-increasing duration of successive protostellar classes, where Class 0 (with 248 M⊕ dust mass) lasts 104 yr, Class I (with 96 M⊕ dust mass) lasts 105 yr, Class II (with 5-15 M⊕ dust mass) lasts 106 yr, and Class III (? dust mass) lasts 107 yr.
Evidence for Kuiper belt objects (KBOs) formed by gravitational/streaming instability
¶ “We have searched 101 Classical trans-Neptunian objects for companions with the Hubble Space Telescope. Of these, at least 21 are binary. The heliocentric inclinations of the objects we observed range from 0.6-34°. We find a very strong anticorrelation of binaries with inclination. Of the 58 targets that have inclinations of less than 5.5°, 17 are binary, a binary fraction of 29+7-6 %. All 17 are similar-brightness systems. On the contrary, only 4 of the 42 objects with inclinations greater than 5.5° have satellites and only 1 of these is a similar-brightness binary. This striking dichotomy appears to agree with other indications that the low eccentricity, non-resonant Classical trans-Neptunian objects include two overlapping populations with significantly different physical properties and dynamical histories.”
(Noll et al. 2008)
¶ “The 100 km class binary KBOs identified so far are widely separated and their components are similar in size. These properties defy standard ideas about processes of binary formation involving collisional and rotational disruption, debris re-accretion, and tidal evolution of satellite orbits
(Stevenson et al. 1986).” “The observed color distribution of binary KBOs can be easily understood if KBOs formed by GI [gravitational instability].” “We envision a situation in which the excess of angular momentum in a gravitationally collapsing swarm prevents formation of a solitary object. Instead, a binary with large specific angular momentum forms from local solids, implying identical composition (and colors) of the binary components.”
(Nesvorny et al. 2010)
………………..
Hybrid accretion planets and moons:
¶ Hybrid accretion is a working hypothesis for the formation of multiplicities of well-behaved super-Earths in stellar systems and similar multiplicities of well-behaved moons around giant planets. The evidence for the inside-out formation multiplicities of hybrid accretion objects is particularly tentative.
¶ An alternative planet formation mechanism combines the formation of planetesimals by streaming instability with the hierarchical accretion of these planetesimals into super-Earths or hybrid-accretion moons, hence ‘hybrid’.
¶ This hybrid mechanism for planet formation was first proposed for the formation of gas giant planets by Thayne Curie (2005). Alternatively, the mechanism is suggested here for the formation of
multiplicities of super-Earths, designated as ‘cascades’ of super-Earths.
¶ Gas in accretion disks rotates slower than the Keplerian rate, due to gas pressure, and this sub-Keplerian rotation of gas creates a drag on dust grains, causing dust to spiral inward toward the inner edge or nearest gap in accretion disks, where the resulting concentration of dust can result in a form of gravitational instability known as streaming instability.
¶ Planetesimals (of indeterminate size) form by streaming instability, presumably at the inner edge of the protoplanetary disk, whose inner edge may be defined by the magnetic corotation radius of its young stellar object (YSO). A myriad of planetesimals thus formed merge by gravitational accretion until the largest hierarchical component clears its orbit at a nominal super-Earth mass. Orbit clearing by the anchor super-Earth precludes further planetesimal formation by streaming instability against the magnetic corotation radius of the YSO, but it begins the concentration of dust grains against the strongest outer resonances of the anchor super-Earth.
¶ Dust grains accumulate in the accretion disk dead zone aganist the strongest outer mean-motion resonance of the anchor super-Earth, forming a second-generation of planetesimals by streaming instability that similarly accrete to form a second-generation super-Earth. In this manner, a cascade of super-Earths may form sequentially from the inside out.
¶ Giant planets may also form hybrid-accretion moons around giant planets. The 5 planemo moons of Uranus; Miranda, Ariel, Umbriel, Titania and Oberon, are perhaps the best example of a moony hybrid-accretion cascade in our solar system.
¶ Sourav Chatterjee and Jonathan C. Tan quantified this form of inside-out planet formation mechanism in a more general form, encompassing either pebble accretion or ∼1 M⊕ planet formation by gravitational instability. “Formation of a series of super-Earth mass planets from pebbles could require initial protoplanetary disks extending to ∼100 AU.” (Chatterjee and Tan 2013)
¶ In cascades of super-Earths, the outermost planetary pair typically exhibits a greater period ratio than the other adjacent planetary pairs in the same stellar system. This may lend support to inside-out formation of super-Earth cascades, if each outer super-Earth in turn experiences a degree of inward migration due to the inertia of the accretion disk pressing in on its outer mean-motion resonances, where the inward inertia of the accretion disk is due to the general spiraling in of the accretion disk due to the continuous outward transfer of angular momentum. The outermost super-Earth in a cascade, however, may be somewhat shielded from this effect, since the accretion disk dissipates before another tranche of planetesimals can form still another super-Earth. See Table 1 for a comparison of the period ratios for two stellar systems.
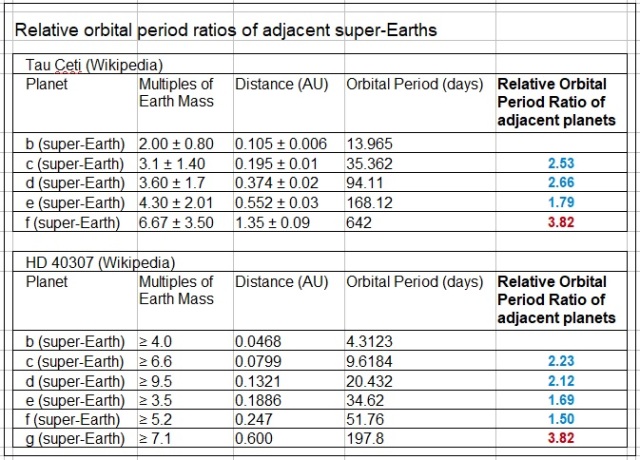
Table 1. Orbital period ratios of adjacent super-Earths. Note the greater orbital period ratio between the outermost adjacent super-Earth pairs (red) compared to inner adjacent super-Earth pairs (blue).
………………..
Flip-Flop Fragmentation (FFF):
¶
Terminology:
¶ For the purposes of this paper, ‘protoplanetary disk’, or ‘proto-disk’, will refer to both the traditional circumstellar disks associated with young stellar objects as well as hypothesized accretion disks around prestellar cores prior to the formation of the first hydrostatic core. This extended definition allows for a more inclusive discussion of the early stages of disk formation and evolution. Likewise, YSO will be inclusive of prestellar and protostellar systems.
¶ Also, ‘disk instability’ or ‘proto-disk instability’ or ‘pseudo-disk instability’ is used interchangeably with ‘asymmetrical FFF’.
¶ Planet formation theory is constrained by the mass and evolution of protoplanetary disks. A recent study by (Tychoniec et al., 2018) made a counterintuitive discovery that found decreasing accretion disk mass with protostellar evolution. The study measured a dramatic decrease in dust mass with increasing protostellar age, where measured dust mass is assumed to be a proxy for overall accretion disk mass. Disk dust mass was measured to decrease from 248 M⊕ in Class 0 protostars, to 96 M⊕ in Class I protostars, to 5-15 M⊕ in Class II protostars. And if the youngest (Class 0) protostars have the most massive disks, then it’s a reasonable extrapolation that still-younger prestellar objects may have still more-massive disks. If protostars are steadily accreting while their surrounding accretion disks are rapidly dissipating, then these opposing trends suggest an early crossover point, before which accretion disks should be much-more massive than their diminutive, planetary-mass prestellar/protostellar cores, resulting in dynamically-unstable systems.
¶ “The compact components around the Class 0 protostars could be the precursors to these Keplerian disks. However, it is unlikely that such massive rotationally supported disks could be stably supported given the expected low stellar mass for the Class 0 protostars: they should be prone to fragmentation”. (Li et al. 2014)
¶ ‘Flip-flop fragmentation’ (FFF) is an alternative hypothesis for the formation of stars with gaseous satellites, in the form of gas-giant planets and larger brown and red dwarf companions. FFF suggests that gas giant planets (not formed by trifurcation) originated as prestellar/protostellar cores of stellar systems, and their stellar hosts formed by disk instability of stellar-mass proto-disks or pseudo-disks, making gas-giant planets and companions the progenitors of their stellar systems, and older than their much-more-massive stellar hosts.
¶ Proto-disks are supported by Keplerian rotation, with their outer boundaries defined by the transition to pressure-supported pseudo-disks beyond. The outer boundary of a pseudo-disk is called the centrifugal radius, which is where material from the collapsing envelope falls on the disk, and its inner boundry transitions to the protoplanetary disk. The envelope feeds the pseudo-disk and the pseudo-disk feeds the protoplanetary disk, and if angular momentum is conserved in the inward cascaded of matter, then the specific angular momentum of pseudo-disks should be similar to that of their associated protoplanetary disks.
¶ FFF of stellar-mass pseudo-/proto-disks surrounding diminutive prestellar or protostellar objects may occur by means of (spiral) density waves, where the mode of the density wave dictates the type of disk instability, forming either solitary disk instability (d-i) objects by asymmetrical FFF or twin d-i objects by symmetrical FFF.
Asymmetrical FFF:
¶ A Keplerian disk in orbital freefall may be more consistent with the gravitational interactions of a many-body system, such as a globular cluster, than a pressure-supported gaseous system, like a gas globule in virial equilibrium, thus exhibiting negative heat capacity like globular clusters. In systems with negative heat capacity, entropy increases when the mass is more centrally concentrated, and to the extent that thermodynamic evolution prefers catastrophism over gradualism, this means (gravitational) disk instability.
¶ Asymmetrical (m = 1 mode) density waves in stellar-mass disks around diminutive prestellar/protostellar cores are suggested to form solitary d-i objects that are necessarily much-more massive than their diminutive cores. When an overlying disk greatly exceeds the mass of its prestellar/protostellar core, the disk has inertial dominance of the system, where the diminutive core is unable to damp down disk inhomogeneities from amplifying into runaway disk instability. Asymmetrical FFF breaks the radial symmetry of the system and catastrophically projecting mass inward by shifting the center of mass of the system toward the nascent d-i object, with the secondary effect of injecting the diminutive prestellar/protostellar (pre/protostellar) core into a planetary satellite orbit around the d-i object.
¶ A Hot Jupiter orbiting its host star in a low, hot orbit has a much-lower specific angular momentum than its precursor pre/protostellar system, where the pre/protostellar system constituted a high-angular-momentum disk orbiting a diminutive pre/protostellar core. The clumping attendant in asymmetrical FFF converts the high-angular-momentum disk into a low-angular-momentum star, and the injection of a diminutive pre/protostellar core into a low hot orbit does not contribute much toward the specific angular momentum of the mature system. Asymmetrical FFF is a catastrophic process for projecting mass inward, and as such it similarly requires a catastrophic mechanism for projecting angular momentum outward. Bipolar outflows are the likely mechanism for sloughing off angular momentum in catastrophic disk instability, because outflows are scalable to a catastrophic rate of gravitational collapse, unlike the gradual angular momentum redistribution resulting from viscous accretion, where the rate of infall from the proto-disk on the stellar core is limited by the extent shear forces.
Hot-cold Jupiter divide:
¶ Asymmetrical FFF is suggested to be the origin of gas-giant planets not formed by trifurcation, from mini-Neptunes at the low-mass end, likely up to low-mass brown dwarfs at the high-mass end. Objects formed by asymmetrical FFF are divided into two camps by orbital period, nominally designated hot and cold Jupiters, with a 10–100 d orbital-period valley in between. The orbital period valley separating the hot-and-cold distributions indicates 2 discrete reservoirs, with bimodal specific angular momentum.
¶ Disks around pre/protostellar cores are composed of two parts, a flat inner proto-disk and a more-diffuse outer pseudo-disk. Inner proto-disks are supported by Keplerian rotation, and their outer boundaries are defined by the transition to pressure-supported pseudo-disks beyond. The outer boundary of a pseudo-disk is called the centrifugal radius, which is where material from the collapsing envelope falls on the disk, and its inner boundary transitions to the protoplanetary disk. The envelope feeds the pseudo-disk and the pseudo-disk feeds the proto-disk disk and if angular momentum is conserved in the transitions, then the specific angular momentum of pseudo-disks should be similar to that of their associated proto-disks. Bimodal populations of gas-giant planets suggest formation by asymmetrical FFF from separate reservoirs, namely, pseudo-disks and proto-disks, but which reservoir creates hot Jupiters and which creates cold Jupiters?
¶ The sharply-defined planetary desert in Fig. 2 is defined by the two dashed line segments that meet at a right angle. The high definition of this planetary desert suggests that they hail from the disk with the highest definition, which is the proto-disk. If so, then cold jupiters presumably form by asymmetrical FFF of the more-distant and more-nebulous pseudo-disk. The larger average size of cold Jupiters may suggest that proto-disks dissipate faster than pseudo-disks. But typically less-massive hot Jupiters are hosted by typically more-massive host stars, which may or may not be indicative of relative disk mass. Hot Jupiters are also found around stars with elevated metallicity, compared to the stars hosting cold Jupiters, which could mean that proto-disks have a higher metallicity than their pseudo-disk counterparts, or could merely mean that proto-disk instability requires elevated metallicity to occur.
¶ Hot Jupiters tend to be solitary planets, whereas cold Jupiters are more often found in multiple planet systems. This appears to indicate that pseudo-disks can be regenerated from the surrounding envelope and undergo repeated instances of pseudo-disk instability, whereas proto-disk instability may end the FFF phase of YSOs.
¶ The similar specific angular momentum of pseudo-disks and proto-disks should cause a nascent pseudo-disk d-i object to merge with its proto-disk, such that the stellar-mass pseudo-disk d-i objects steals the proto-disk from the planetary-mass prestellar core, injecting the prestellar core into a high cold planetary orbit.
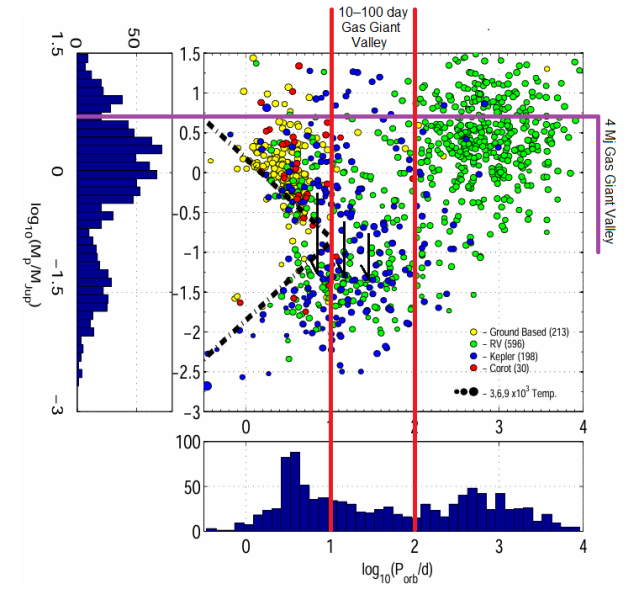
Figure 2: Log-log plot of planetary mass as a function of orbital period:
– Red lines: Relative desert of giant planets with an orbital period of 10–100 days, separating hot Jupiters from cold Jupiters
– Purple line: Relative desert of giant planets with a mass of 4 Mj
– Black dashed line segments, meeting at a right angle: outlines the planetary desert
– Black lines with arrowheads: indicates formational mass and resulting mass
Image credit: Modified from Mazeh, Holczer and Faigler, A&A 589, A75 (2016), Fig.1
Neptune-, Saturn-, and Jupiter-mass planets in relation to the planet desert:
¶ Neptune-mass planets in the range of 10–30 M⊕ not formed by trifurcation presumably formed as very-early prestellar cores, predominantly composed of dust and ice. Interstellar gas, composed chiefly of hydrogen and helium, experiences a degree of pressure support even during Jeans instability freefall, such that dust always freefalls faster than gas. During freefall, the extent to which volatiles condense and freeze on dust grains (increasing their mass) increases their terminal velocity in freefall. Thus the dust and ice at the center of rotation of prestellar systems should have a high ice component. In the ~100,000 year freefall phase of YSOs, the freefall of icy dust may sufficiently outpace the freefall of gas to form a planetary-mass rocky-icy core at the center of rotation, before the gas in the core before the gas in the nascent core becomes sufficiently dense to become opaque to infrared light. These 10–30 M⊕ planets formed by asymmetrical FFF are presumably the origin of Neptune-mass planets.
¶ The relative prevalence of Neptune-mass planets compared to Saturn-mass planets (as apparent in Fig. 2) suggests that Saturn-mass planets formed by asymmetrical FFF may be vulnerable to losing their pithy gaseous component and dissipating back to their Neptune-mass icy-rocky cores in the upheaval of asymmetrical FFF of pseudo-disks. The red arrows in Fig. 2 indicate the suggested Saturn-mass origin of some of the Neptune-mass planets in the 10–100 d orbital period valley prior to losing their gaseous component, suggesting that Saturn-mass planets are not as formationally scarce as they are observationally scarce.
¶ Presumably proto-disks form hot Jupiters and pseudo-disks form cold Jupiters, and the typically solitary nature of hot Jupiters indicates that proto-disk instability typically ends the FFF phase of solar system formation. Moving up and around the planetary desert parameter space in Fig. 2, from Neptune-mass planets through Saturn-mass planets to hot Jupiters, represents increasing age of prestellar systems, with Saturn-mass planets having the highest orbital periods. The perimeter of the planetary desert is traced by two dashed line segments that meet at a right angle in Fig. 2. The right-angle intersection occurs at a planetary mass of about 18% of a Jupiter mass, which is nominally a ‘Saturn’ mass.
¶ In Keplerian systems such as proto-disks, increasing radial distance corresponds to increasing specific angular momentum. But mature, solitary hot Jupiter systems retain only a tiny portion of the angular momentum of the angular momentum of their prestellar proto-disks, with that tiny portion being designated, ‘residual angular momentum’, with the lion’s share of the residual angular momentum vested in the FFF planet. The vast majority of the angular momentum presumably leaves the system in bipolar outflows concurrent with asymmetrical FFF.
¶ Assuming the radial distance to a d-i object is proportional to its proto-disk mass (more-massive proto-disks form more-distant d-i objects), and assuming the residual specific angular momentum is proportional to the radial distance of the d-i object, then the residual specific angular momentum is proportional to the proto-disk mass at asymmetrical FFF. This suggests that prestellar disks reach their maximum mass when the prestellar core has a Saturn mass. Thus, when proto-disks are waxing in mass, their prestellar cores weigh in at mini-Neptune- to Saturn-mass planets, and when proto-disks are waning in mass, their prestellar cores weigh in at Saturn- to hot Jupiter-mass planets.
¶ Presumably, the prestellar core had to achieve a minimal Neptune mass, a ‘mini-Neptune’ mass, before the pseudo-disk was of sufficient size to undergo asymmetrical FFF. Nominally, planets smaller than mini-Neptunes are super-Earths, but there’s likely some overlap in mass and radius between super-Earths formed by hybrid accretion and mini-Neptunes formed by asymmetrical FFF of proto-disks.
Symmetrical FFF:
¶ Symmetrical (m = 2 mode) density waves in massive accretion disks around diminutive prestellar/protostellar cores are suggested to ‘condense’ twin-binary d-i objects, which are much-more massive than their diminutive stellar core. In symmetrical FFF, the twin d-i objects create a bilateral symmetry that does not inertially displace the stellar core from the center of mass, as in asymmetrical FFF, but twin stellar masses orbiting a planetary-mass core constitutes a nonhierarchical system that is (thermo)dynamically unstable. Hierarchy is gradually achieved by orbital interplay, which increases entropy by equipartition of kinetic energy in orbital close encounters. The diminutive stellar core is progressively ‘evaporated’ into a circumbinary orbit around the twin-binary stellar pair, while the binary pair spiral in to conserve system energy and angular momentum, possibly to the point of merging in a luminous red nova (as in our solar system).
¶ There’s insufficient data from binary stars to speculate on whether symmetrical FFF occurs to pseudo-disks, or proto-disks or both. Our solar system and its closest neighbor are suggested to have undergone symmetrical FFF, so symmetrical FFF cannot be uncommon. Our solar system additionally underwent 4 generations of trifurcation, which appears to be the exception, while in the Alpha Centauri system at twice the mass, Proxima did not trifurcate, which appears to be the rule.
¶ Additionally, asymmetrical FFF may be the origin of similar-sized and similar-color binary pairs in the Kuiper belt. And the Pluto system may even have undergone 2 generations of trifurcation, with Nix-Hydra as 1st-generation trifurcation twins and Styx-Kerberos as 2nd-generation twins. And FFF may even explain large accretional galaxies, with spiral galaxies formed by asymmetrical FFF and elliptical galaxies formed by symmetrical FFF, after the twin-binary galaxies have merged. If so, the relative locations of elliptical and spiral galaxies may be indicative. Elliptical galaxies are preferentially found in galaxy clusters, whereas spiral galaxies are more common elsewhere. This suggests that symmetrical FFF may predominate in regions with strong gravitational gradients like galaxy clusters, explaining elliptical galaxies, and in streaming instabilities within solar systems, explaining binary KBOs. And asymmetrical FFF may predominate in regions with low gravitational gradients, explaining spiral galaxies and most systems with gas giant planets.
¶ The brown-dwarf-mass former stellar core in our solar system (Brown Dwarf) and Proxima in the Alpha Centauri system appear to suggest that symmetrical FFF is delayed, compared to asymmetrical FFF, allowing protostellar cores to reach brown dwarf or red dwarf mass prior to symmetrical FFF, but this would also require a longer lived accretion disk than asymmetrical systems appear to indicate. Alternatively, symmetrical FFF may occur just as early as in asymmetrical FFF, but the bilateral symmetry inherent in symmetrical FFF may allow sufficient continued accretion of the pre/protostellar core to reach a brown dwarf or red dwarf mass, before orbital interplay completely disrupts the accretion disk.
4 Jupiter mass (Mj) giant-planet valley:
¶ A recent discovery (Santos et al. 2017) finds a relative scarcity (valley) of gas-giant exoplanets of about 4 Mj. A 4 Mj valley clearly extends across the cold Jupiter population, but the data points are too sparse in the hot Jupiter region and in the 10–100 d orbital period valley to know if the 4 Mj valley extends all the way to the left of the graph in Fig. 2.
¶ The most noteworthy occurrence in the prestellar phase is the formation of the first hydrostatic core (FHSC), which marks the transition from prestellar to protostellar; however, the mass of the FHSC is not well constrained, neither observationally nor theoretically, so the the conjecture that this valley corresponds to the mass of the FHSC is pragmatic. The appearance of the pithy first hydrostatic core (FHSC) at the end of the prestellar phase is suggested here to provide a viscous mechanism for physically impeding disk instability. As gas falls onto a prestellar core, its potential energy is radiated away as infrared photons, but when the prestellar core reaches a critical density, the gas temperature begins to rise, creating hydrostatic gas pressure, which supports the gas against gravitational collapse, forming the FHSC. Gas infalling onto the hydrostatic core creates a shock front which extends out to radii on the order of ~5–10 AU (Tsitali et al. 2013) that may viscously engage with the proto-disk, presumably damping down the positive disk-core feedback necessary for runaway proto-disk instability.
¶ In proto-disks, however, the disk has sufficiently dissipated by the appearance of the FHSC that proto-disk instability no longer occurs. But if pseudo-disks do indeed form cold Jupiters, then it would appear that viscous engagement of a FHSC with its proto disk is sufficient to cause this observed hiatus in pseudo-disk instability. This brief hiatus in pseudo-disk instability may be an indirect effect, wherein FHSC engagement with the proto-disk may damp down density waves in the proto-disk, which may in turn damp down density waves in the pseudo-disk.
¶ The 4 Mj valley, due to the pithiness of the FHSC, may be the best evidence for giant planet formation by asymmetrical FFF.
L1448 IRS3B (See Figure 1):
¶ L1448 IRS3B is suggested here to have formed by symmetrical FFF. This triple star system is composed of a similar-sized binary pair, IRS3B-a & IRS3B-b, with a combined mass of ~1 M☉ in a 61 AU binary orbit, and a distant tertiary companion, IRS3B-c, with a minimum mass of ~0.085 M☉ in a 183 AU circumbinary orbit around the binary pair. This system may become more hierarchical over time, coming to resemble the Alpha Centauri system at half the mass. “Thus we expect the [L1448 IRS3B] orbits to evolve on rapid timescales (with respect to the expected stellar lifetime), especially as the disk dissipates. A natural outcome of this dynamical instability is the formation of a more hierarchical system with a tighter (few AU) inner pair and wider (100s to 1,000s AU) tertiary, consistent with observed triple systems.” (Tobin et al. 2016)
¶ The tertiary protostar, IRS3B-c, is embedded in a spiral arm of the outer disk, with an estimated spiral arm mass of 0.3 M☉. The standard model of companion star formation, expressed by Tobin the Tobin paper, suggests that IRS3B-c formed in situ by gravitational instability from the spiral disk, making IRS3B-c younger than IRS3B-a & IRS3B-b; however, IRS3B-c is brighter at 1.3 mm and 8 mm than its much more massive siblings, as is apparent in Figure 1. Alternatively, a brighter, more-evolved tertiary companion supports formation by symmetrical FFF, with protostar luminosity as a function of age, making the diminutive companion the progenitor of the younger, larger binary pair.
……………….
Trifurcation by centrifugal fragmentation:
¶ First-generation trifurcation is the centrifugal fragmentation of a protostellar core by orbital close encounters with a much-more massive binary stellar pair formed by symmetrical FFF (disk instability) from a massive accretion disk. Trifurcation implies centrifugal fragmentation into 3 components, forming a trinary subsystem that locally decreases the subsystem entropy.
¶ Symmetrical FFF creates a dynamically-unstable trinary system, which is resolved by orbital interplay into a stable hierarchical system, but centrifugal-fragmentation (trifurcation) may occur as during orbital interplay.
¶ In a high angular momentum pre/protostellar system in which the accretion disk is much more massive than its diminutive stellar core, the disk has inertial dominance of the system, which promotes disk-instability fragmentation at a stellar Jeans-mass scale. The type of disk instability fragmentation may depend on the mode of a (spiral) density wave resident in the accretion disk, with asymmetrical (m = 1 mode) density waves collapsing to form solitary disk instability (d-i) objects in a process designated ‘asymmetrical FFF’, and with symmetrical (m = 2 mode) density waves collapsing to form twin d-i objects in a process designated symmetrical FFF.
¶ Asymmetrical FFF inertially displaces the stellar core from the center of mass of the system as the system becomes progressively more asymmetrical during the incipient disk instability, but symmetrical FFF preserves bilateral symmetry of the system, with the protostellar core remaining at the center of the system; however, the much-greater overlying mass of the twin d-i objects is dynamically unstable, resulting in subsequent chaotic orbital interplay that progressively projects mass inward.
¶ In close orbital encounters between objects with dissimilar masses, the less-massive component receives an energy kick at the expense of the more-massive component by the principle of equipartition of kinetic energy, which is the principle used to extract orbital energy from planets by interplanetary spacecraft, known as ‘gravitational slingshot’ or ‘gravity assist’, where the spacecraft is parasitizing the orbital energy of the planet by means of a gravitational interaction.
¶ In addition to this kinetic energy kick, equipartition in close orbital encounters is suggested here to also transfer rotational energy to the stellar core, causing a rotational ‘spin up’ of the core.
¶ Rotational spin causes a spherical core to distort into an oblate sphere. Additional spin up may cause distortion into a triaxial Jacobi ellipsoid, and finally into a bar-mode instability with pinwheel-like tails trailing from the 2 ends of the bar, lagging in rotation. The centrifugal failure mode of a bar-mode instability is suggested here to be trifurcation into three components, wherein the opposing ends of the bar pinch off into twin gravitationally-bound Roche spheres in orbit around the diminutive residual core at the center of gravity and rotation. (The pinwheel tails may constitute 2 additional fragments that form into oversized ‘trifurcation moons’, which will be discussed in the following section entitled, ‘Moons’.)
¶ At the moment of trifurcation, the trifurcated trinary components resemble a smaller (Mini-Me) version of the original symmetrical FFF system, with a massive twin-binary pair orbiting a diminutive core. And like symmetrical FFF, the trifurcated trinary components constitute a dynamically unstable system that’s resolved by orbital interplay, with accompanying spin up of the residual core, potentially resulting in next-generation trifurcation.
¶ Thus, trifurcation of a stellar core following symmetrical FFF fosters next-generation trifurcation, and etc., potentially creating a cascade of successively-smaller binary pairs, like Russian nesting dolls. Our solar system retained 3 of the 4 sets of twin binary pairs from 4 generations of trifurcation, namely Jupiter-Saturn, Uranus-Neptune and Venus-Earth.
¶ Trinary star systems with diminutive companion stars orbiting similar-sized twin-binary pairs, such as Alpha Centauri and L1448 IRS3B, are also suggested to have formed by symmetrical FFF, but without subsequent trifurcation. The companion star, Proxima Centauri, is the presumed former stellar core of the Alpha Centauri system, but Proxima may have been too massive in relation to Alpha Centauri A and B to have trifurcated, or alternatively, the specific angular momentum of the Alpha Centauri system may have been too high for trifurcation.
¶ Stellar systems supported by Keplerian rotation exhibit negative heat capacity, where entropy increases as the mass becomes more centrally concentrated. Equipartition of kinetic energy tends to equalize kinetic energy in orbital close encounters, resulting in differential velocity between objects with disparate mass, causing more-massive objects to spiral in and less-massive objects to spiral out, which is the mechanism of core collapse in globular clusters, and the evolution toward hierarchy in stellar systems.
¶ Stellar systems formed by symmetrical FFF are born nonhierarchical, and similarly newly trifurcated subsystems are also born nonhierarchical. Subsequent thermodynamic evolution causes mass to sink inward, transforming dynamically-unstable nonhierarchical systems and subsystems into stable hierarchical systems over time.
¶ Thermodynamic evolution toward equipartition of kinetic energy appears to require a rotational component in order to simultaneously conserve both energy and angular momentum. Scheeres et al. (2000) calculates that the rotation rate of asteroids tends to increase in (prograde) gravitational encounters with other asteroids and planets, providing evidence for the rotational spin up underpinning trifurcation.
Why trifurcation and not bifurcation:
¶ A flywheel on a piece of machinery spun up to the point of centrifugal failure will shatter in an unpredictable fashion, but in the spin up of a planetary-mass, the local, gravitational cohesive force presumably enforces symmetry by preventing subfragmentation.
¶ If the spinning object were a glob of mercury held together by surface tension, then whatever shape provided the lowest total surface area (while conserving angular momentum) would be favored. A triaxial Jacobi ellipsoid has a lower surface area than its trifurcated self, so trifurcation presumably cannot proceed until the core spins up into a distorted bar-mode instability, with the ends of the bar thickened like a bow tie. Bifurcation into twin objects would have a still-lower surface area than trifurcation into 3 objects, but catastrophic trifurcation occurs on the way to bifurcation, where centrifugal fragmentation occurs as soon as it is thermodynamically favored, resulting in a trinary residual core. The physics of gravitationally-bound objects are not a perfect analogy to the surface tension in globules of mercury, but the effects should be similar.
Dynamical Bar-mode Instability
¶ Our solar system apparently formed by symmetrical FFF, followed by 4 generations of trifurcation, which created 1 + 4 = 5 sets of binary pairs (binary-Sun, binary-Companion, Jupiter-Saturn, Uranus-Neptune, and Venus-Earth + residual core Mercury):
– 1st-gen trifurcation of Brown Dwarf (protostellar core) by binary-Sun yields, binary-Companion + SUPER-Jupiter (residual core)
– 2nd-gen trifurcation of SUPER-Jupiter by binary-Companion yields, Jupiter-Saturn + SUPER-Neptune (residual core)
– 3rd-gen trifurcation of SUPER-Neptune by Jupiter-Saturn yields, Uranus-Neptune + SUPER-Earth (residual core)
– 4th-gen trifurcation of SUPER-Earth by Uranus-Neptune yields, Venus-Earth + Mercury (residual core)
(Note, unorthodox capitalization indicates unorthodox definitions. ‘SUPER-Jupiter’, ‘SUPER-Neptune’ and ‘SUPER-Earth’ are the names for the former residual cores formed in the first three trifurcation generations. And ‘Brown Dwarf’ is the name of the original protostellar core of the solar system.)
Trifurcation as a fractionation process:
¶ Trifurcation is presumably a fractionation process, which is particularly manifest if the trifurcating core were internally differentiated, such that low-density volatile components are preferentially centrifugally slung into the bar-mode arms, while the denser material is preferentially left behind in the residual core. If prestellar cores do indeed begin with the formation of Neptune-mass icy-rocky cores formed by dust and ice sedimentation prior to the appearance of the FHSC, then our former protostellar core, Brown Dwarf, was indeed internally differentiated and likely possessed an iron inner core. Due to fractionation, each next-generation trifurcation-pair had a denser composition than its proceeding generation, and the densest composition of all was the residual core of the 4-th generation trifurcation, namely Mercury, which has a proportionately-larger iron-nickel core than its twin-binary siblings, Venus and Earth.
¶ Fractionation during trifurcation is presumably isotopic as well, likely explaining Jupiter’s elevated deuterium-to-hydrogen (D/H) ratio, which is about twice primordial, resulting from fractionation during 1st-generation trifurcation, where binary-Companion became depleted in deuterium. Mass-dependent fractionation of elements with 3 stable isotopes, like oxygen, creates a fractionation line, where mass fractionation merely moves the data point along the fractionation line. Objects that lie on different fractionation lines were formed from different reservoirs, while objects formed from the same well-mixed reservoir will all lie on the same fractionation line. Thus, all the trifurcation products of our solar system are direct descendants of Brown Dwarf and should all lie on the 3-oxygen-isotope ‘Brown Dwarf fractionation line’, which we know as the ‘terrestrial fractionation line’. Mars is not a trifurcation planet and indeed we know that it does not lie on the terrestrial fractionation line.
Three debris disks formed by trifurcation and secular evolution, following symmetrical FFF:
1) Trifurcation debris disk, > 4,567 Ma
2) Solar-merger debris disk, at 4,567 Ma
3) Companion-merger debris disk, at 650 Ma
¶ Our solar system began with a protoplanetary disk, which presumably formed Mars by hybrid accretion.
¶ Trifurcation is apparently a messy process. Four generations of trifurcation following symmetrical FFF created a trifurcation debris disk prior to 4,567 Ma, which was siderophile depleted (because the trifurcated objects were internally differentiated) and lay on the 3-oxygen-isotope terrestrial fractionation line. The trifurcation debris disk formed the hot classical KBOs.
¶ Binary-Sun spiraled in and merged at 4,567 Ma, forming the solar-merger debris disk with live stellar-merger-nucleosynthesis short-lived radionuclides (26Al, 60Fe) and alpha-element enrichments (12C, 16O). The solar merger debris disk formed asteroids and chondrites in the asteroid belt.
¶ Binary-Companion spiraled in and merged at 650 Ma, forming the Companion-merger debris disk that lay on the 3-oxygen-isotope terrestrial fractionation line but was not siderophile depleted. The Companion-merger debris disk formed the cold-classical KBOs.
¶ Two principles are involved in transferring energy and angular momentum between 2 sets of binary pairs of differing mass, the first being the principle of resonance chasing, which attempts to synchronize orbits to simple integer ratios, designated ‘resonance chasing’, and the second being the principle of equipartition of kinetic energy, which attempts to equalize kinetic energy in orbiting objects of disparate mass, designated ‘orbit inflation’.
¶ Secular evolution in star systems by means of equipartition of kinetic energy increases the entropy of the system, which results in thermodynamic evolution by projecting mass inward by projecting angular momentum outward. In a stellar system context, imagine a central binary star with a binary planet in a circumbinary orbit around the binary star. In such a system, system entropy increases when the binary planet climbs to a higher circumbinary orbit, causing the binary stellar components to spiral in. And system entropy also increases when the planetary components of the binary planet spiral out from their common barycenter, also causing the binary stellar components to spiral in. For the sake of clarity, the terms
– ‘Type 1 orbit inflation’, and
– ‘Type 2 orbit inflation
will be defined to differentiate these 2 thermodynamically similar effects.
Type 1 orbit inflation:
¶ Type 1 orbit inflation will be defined as progressively increasing the semi-major axis of a circumbinary orbit by perturbation of the central binary pair, causing the circumbinary object to spiral out from the central binary pair. In the case of trifurcation, the circumbinary ‘object’ subject to type 1 orbit inflation will typically be a gravitationally-bound trinary orbital system, composed of a twin binary pair and a residual core, or the ‘object’ could be a gravitationally-bound assemblage of multiple trifurcation generations. Type 1 orbit inflation unwinds trifurcation generations, causing a circumbinary object to spiral out and escape its circumbinary orbit by exceeding the Hill sphere of the binary pair.
¶ Following the 4th-generation trifurcation, Venus-Earth-Mercury were in a circumbinary orbit around the binary pair Uranus-Neptune. Type 1 orbit inflation caused Venus-Earth-Mercury to spiral out until they escaped the Uranus-Neptune Hill sphere to be captured by Jupiter-Saturn, while Jupiter-Saturn was still within the binary-Companion Hill sphere. Uranus-Neptune spiraled out from Jupiter-Saturn to be captured by binary-Companion. Continued type 1 orbit inflation caused Uranus-Neptune to escape from the binary-Companion Hill sphere to be captured by binary-Sun via the (outer) L2 Lagrangian point of binary-Companion, injecting the binary pair Uranus-Neptune into a higher heliocentric orbit than binary-Companion.
¶ Following the loss of Uranus-Neptune, Jupiter-Saturn, with Venus-Earth-Mercury in tow, spiraled out from binary-Companion until it was captured by binary-Sun via the (inner) L1 Lagrangian point, injecting Jupiter-Saturn into a lower heliocentric orbit than binary-Companion. Next, Venus-Earth-Mercury spiraled out from Jupiter-Saturn until the trinary grouping was captured by binary-Sun via the inner L1 Lagrangian point of Jupiter-Saturn.
¶ Finally, type 1 orbit inflation caused Mercury to spiral out from Venus-Earth to be captured into a heliocentric orbit via the (inner) L1 Lagrangian point, injecting Mercury into a lower heliocentric orbit than Venus-Earth.
¶ Imagine Uranus-Neptune spiraling out from binary-Companion, powered by Type 1 orbit inflation. Capture of Uranus-Neptune by binary-Sun presumably occurred when the 3 components (binary-Sun, binary-Companion, and Uranus-Neptune) were in alignment, with Uranus-Neptune at the (outer) L2 Lagrangian point of binary-Companion. At L2, the prograde (CCW) rotation of Uranus-Neptune around binary-Companion added to the prograde rotation of binary-Companion around the Sun, giving Uranus-Neptune a prograde kick into a higher orbit.
¶ By comparison, imagine Jupiter-Saturn spiraling out from binary-Companion, powered by Type 1 orbit inflation. Capture of Jupiter-Saturn by binary-Sun presumably occurred when the 3 components (binary-Sun, Jupiter-Saturn, and binary-Companion) were in alignment, with Jupiter-Saturn at the (inner) L1 Lagrangian point of binary-Companion. At L1, the prograde (CCW) rotation of Uranus-Neptune around binary-Companion was 180° opposed to the prograde (CCW) rotation of binary-Companion around the Sun, giving Uranus-Neptune a retrograde kick into a lower orbit.
¶ The energy necessary to induce Jupiter-Saturn and Uranus-Neptune to spiral out from binary-Companion came from the gravitational potential energy of the binary components of binary-Companion, causing binary-Companion components to spiral in. And following binary-Sun merger at 4,567 Ma, binary-Companion experienced a variation of type 1 orbit inflation causing the binary components to continue to spiral in due to perturbation by the Sun. The potential energy transfer from its binary components to its heliocentric orbit, propelled binary-Companion into a progressively-more eccentric heliocentric orbit over time until the super-Jupiter-mass components ultimately spiraled in and merged at 650 Ma. The rapid unwinding of the trifurcation regime by 4,567 Ma, versus the slow spiral in of the binary components over a time span of almost 4 billion years appears to indicate vastly-greater efficiency of binary-binary perturbation, compared to binary-solitary perturbation.
Type 2 orbit inflation:
¶ Type 2 orbit inflation will be defined as the spiral-out separation of binary pairs by perturbation by a more-massive binary pair. The fact that twin-binary pairs are in adjacent heliocentric orbits today appears to indicate a sequential application of type 1 orbit inflation, followed by type 2 orbit inflation, but instead, the nested structure inherent in multiple trifurcation generations may be the cause. For instance, the energy required for Mercury to spiral out of the Venus-Earth gravity well was derived from the gravitational potential energy of Venus-Earth, causing Venus-Earth to spiral in. Only after Mercury escaped the Venus-Earth Hill sphere and was captured into a heliocentric orbit did Venus-Earth spiral out from their mutual barycenter by means of type 2 orbit inflation.
¶ Jupiter-Saturn, Uranus-Neptune and Venus-Earth spiraled out of twin binary orbits and separated into solitary heliocentric orbits by means of type 2 orbit inflation, presumably prior to 4,567 Ma. Following the capture of Uranus-Neptune and Jupiter-Saturn by binary-Sun by way of type 1 orbit inflation, binary-Companion also experienced type 2 orbital inflation; however, binary-Sun merger occurred before the binary-Companion components separated. Indeed, binary-Companion was the predominant cause of the binary-Sun merger.
¶ Imagine Venus-Earth spiraling out by Type 2 orbit inflation until breaching their ‘binary Hill sphere’. Capture likely occurred when binary-Sun Venus and Earth were aligned, such that Venus was captured via the (inner) binary L1 Lagrangian point and Earth was captured via the (outer) binary L2 Lagrangian point. At the L2 Lagrangian point, the prograde (CCW) binary orbit of Earth around the Venus-Earth barycenter added to the prograde (CCW) heliocentric orbit of Venus-Earth around binary-Sun, giving Earth a forward kick into a higher heliocentric orbit when the pair separated. The opposite effect occurred to Venus at the binary L1 Lagrangian point, where the prograde (CCW) binary orbit of Venus around the Venus-Earth barycenter was 180° opposed to the prograde (CCW) heliocentric orbit of Venus-Earth around binary-Sun, giving Venus a backward kick into a lower heliocentric orbit when the pair separated. And the same thing happened with the other twin-binary pairs.
Resonance chasing:
¶ Equipartition of kinetic energy in globular clusters leads to core collapse, which projects mass inward and angular momentum outward. This form of secular evolution progressively increases system entropy. Binary-binary interactions that similarly project mass inward while conserving energy and angular momentum may take the form of Type 1 or Type 2 orbit inflation, and the energy transfer mechanism that drives orbit inflation is also of interest.
¶ One form of gravitational energy transfer mechanism is suggested here to be resonance chasing, which involves sympathetic gravitational interactions that strive toward mean motion resonances (MMRs).
¶ A mean motion resonance (MMR) occurs when orbital periods are in a simple integer ratio, and resonance chasing causes binary orbital periods to mutually adjust toward the next MMR that is thermodynamically favored, requiring that the orbital period of the more-massive binary pair increases, while the orbital period of the less-massive binary pair decreases.
¶ In addition to extra-binary interactions, internal tidal interactions between the binary components of a binary pair cause secular evolution, causing both high- and low-mass binary pairs to very gradually spiral in, so binary-binary interactions cannot permanently lock in on one MMR. After rapidly evolving to a MMR by resonance chasing, secular evolution slows down until the periods begin to drift away from the MMR in a direction that cannot be reversed due to entropy evolution. Once the tug of the next thermodynamically-favored MMR exceeds the drag of the last MMR, rapid secular evolution by resonance chasing occurs until the next thermodynamically-favored MMR is reached.
¶ The above description is for a highly simplified case where there are only 2 binary pairs that only interact with one another, which would be unusual in a recently trifurcated system with multiple trifurcation generations. So in a recently trifurcated system like our early solar system, resonance chasing may be almost continuous, without the slow down that occurs when a system temporarily locks in on an MMR.
¶ For resonance chasing to also apply to both type 1 orbit inflation, resonance chasing must apply to MMRs between binary orbital periods and circumbinary orbital periods of lower-mass binary pairs.
Pluto system:
¶ Binary asteroids and Kuiper belt objects (KBOs) presumably formed by symmetrical FFF, and some may have undergone trifurcation as well. The Pluto system has 4 small moons that could be characterized as two sets of twin binary pairs in descending size from two trifurcation generations of a former sedimentary core formed at the center of rotation of the streaming instability. Thus, Pluto-Charon would be the d-i twins, with Nix-Hydra (50 × 35 × 33 km), (65 × 45 × 25 km) as 1st–generation trifurcation twins, and Styx-Kerbos (16 × 9 × 8 km), (19 × 10 × 9 km) as 2nd-generation trifurcation twins, but the residual core from the 2nd-generation trifurcation is either missing or more likely below the resolution of the Hubble telescope. This characterization would make the Pluto system a miniature version of our solar system; however, Pluto’s smaller moons are some 30,000 times less massive than Charon, whereas the Sun is only 1000 times the mass of Jupiter, likely pointing to differing dynamics in systems formed by streaming instability.
¶ The elongated shapes of the 4 small moons may be evidence for elongation of a core into an bar-mode-instability, with the trifurcated objects having insufficient mass to subsequently assume hydrostatic equilibrium.
………………..
Trifurcation moons:
¶ Since the iron core of Earth’s Moon is disproportionately small compared to Earth’s iron core, the Moon cannot be the residual core of the 4th-generation trifurcation in our solar system. An alternative origin story is suggested by the visual depiction of bar-mode instabilities in computer models, with spiral arms trailing from the two ends of the central bar. In a bar-mode instability, the bar rotates like a solid object. If the outer ends of the bar rotate with super-Keplerian speed, it may cause material to slough off into twin spiral arms that rise into a slightly-higher orbit to enable Keplerian rotation, which is what appears to happen in computer simulations. The formation of spiral arms in computer simulations suggests that a bar-mode instability held together by gravity cannot simultaneously conserve both energy and angular momentum without forming spiral arms.
¶ Including spiral arms, the rotational spin up of a protostellar core following symmetrical FFF may cause centrifugal fragmentation of the core into 5 components, where the bar trifurcates into a twin pair of trifurcation planets plus a planetary-mass residual core, and where the spiral arms gravitationally pinch off into a pair of ‘trifurcation moons’. So if spin up centrifugal fragmentation of protostellar cores and residual cores indeed forms 5 objects, including two formed from the spiral arms, a more fitting term would be ‘quintifurcation’, which is sufficiently awkward that trifurcation+2 will suffice when including twin trifurcation moons.
Dynamical Bar-mode Instability
¶ ‘Trifurcation moons’ are suggested to form during trifurcation+2 if the spiral arms streaming from the ends of the central bar gravitationally pinch off into their own moony Hill spheres, while the bar is trifurcating. And the resulting twin trifurcation moons remain gravitationally attached to their respective twin planets.
¶ In addition to Earth’s oversized Moon, Titan at Saturn and Triton at Neptune are also suggested to be trifurcation moons, with all other moons as insitu hybrid-accretion moons or insitu streaming instability moons or captured moons.
¶ Trifurcation moons are born with no net angular momentum with respect to their respective their respective twin-binary planets, but the first kick from the host binary pair that induced trifurcation+2 installs one trifurcation moon into a prograde orbit around its respective twin planet, while symmetrically installing its twin trifurcation moon in a retrograde orbit around its respective twin planet. Thus, since Luna acquired a prograde orbit around Earth, we know by symmetry that Venus’ corresponding trifurcation moon acquired a (decaying) retrograde orbit, where retrograde orbits ultimately spiral in and merge with their host planet.
Jupiter-Saturn:
¶ If Titan is Saturn’s prograde trifurcation moon, then Jupiter presumably had a retrograde trifurcation moon that has long since merged with the planet, possibly at 4,562 Ma, forming enstatite chondrites that lie on the 3-oxygen isotope ‘terrestrial fractionation line’, with the moony merger explosion possibly melting water ice in nearby CI chondrites, forming dolomites in internal fissures.
Uranus-Neptune:
¶ The retrograde orbit of Triton at Neptune presupposes a former prograde trifurcation moon at Uranus, which was presumably lost when binary-Companion’s progressively-increasing eccentricity overran Uranus, stripping Uranus of its moons and causing its 98° axial tilt. If any of Uranus’ moons merged with binary-Companion they fogged the solar system and contributed to the Sturtian glaciation of the Cryogenian period. Triton’s retrograde orbit will ultimately spiral in to merge with Neptune in about 3.6 billion years, which may cause another glaciation on Earth.
Venus-Earth:
¶ The prograde orbit of Earth’s Moon, Luna, presupposes a former retrograde trifurcation moon around Venus, which may have spiraled in and merged with Venus at 579 Ma, fogging the inner solar system, causing the Gaskiers glaciation on Earth. See section, ‘Venusian cataclysm’.
Trifurcation moon summary:
– Jupiter: former retrograde trifurcation moon that may have merged with the planet at 4,562 Ma
– Saturn: prograde trifurcation moon Titan
– Uranus: lost prograde trifurcation moon
– Neptune: retrograde trifurcation moon, Triton
– Venus: former retrograde trifurcation moon that may have merged with the planet at 579 Ma
– Earth: prograde trifurcation moon, Luna
¶ Earth’s oversized Moon is much larger in proportion to Earth than Titan is to Saturn, which may be expected, since a rocky planetary core must achieve a mass of 5–10 M⊕ before it can hold onto hydrogen and helium. The 1st-generation residual core, SUPER-Jupiter, was overwhelmingly composed of volatile hydrogen and helium, and since the spiral arms formed in the 2nd-generation trifurcation represented an additional fractionation, the gravitational collapse of the less-volatile components were only able to form a moon the size of Titan.
¶ The rocky residual core of the 3rd-generation trifurcation, SUPER-Earth, had essentially no hydrogen and helium envelope, such that the spiral arms trailing from the outer ends of the central bar were nearly gas free, resulting in a proportionally-more-massive trifurcation moon at Earth compared to Saturn. But the fractionation of the spiral arm trailing from the bar end that became Earth explains the proportionally smaller iron core of the Moon compared to Earth. The size of the Lunar core is only about 20% of the volume of the Moon, in contrast to about 50% for Earth and Venus.
………………..
Former binary-Companion:
¶ The mass of former Brown Dwarf and former binary-Companion may someday be statistically calculated if other extraterrestrial examples of trifurcated stellar systems are identified, and if other symmetrical FFF systems that failed to trifurcate are identified, like the Alpha Centauri system. But if the failure of Proxima Centauri to trifurcate was due to excessive mass of its Proxima Centauri core, then the Alpha Centauri system may not be a good analogy for comparison.
¶ For planetary trifurcation, we only have one residual core for examination, that being Mercury. But all three terrestrial planets were presumably immersed in the brief LRN phase of the binary-solar merger at 4,567 Ma, with Mercury more deeply immersed than Venus, and Venus more deeply immersed than Earth, which presumably stripped away all oceans and likely boiled off an indeterminate quantity of crustal rock as well. Some studies indicate that Venus has a similar-size iron core as Earth in a planet with only 81.5% of Earth’s mass, which could indicate that Venus and Earth, which suggests that Venus may have lost nearly 20% of its crust. And if so, then Mercury lost a still greater percentage.
¶ So the 4th generation trifurcation is tainted by LRN immersion. But even without LRN immersion, planetary accretion theory calculates that a minimum core mass of 5 m⊕ is required to begin hydrogen and helium accretion, such that any hydrogen and helium volatiles contained by the 3rd trifurcation generation residual core (SUPER-Earth) would subsequently have been lost during and following the 4th generation trifurcation. Uranus and Neptune, with considerably greater mass than 5 m⊕ should be largely unaffected by volatile loss following trifurcation, with unknown volatile loss during trifurcation. So, the products of the 2nd (Jupiter & Saturn) and 3rd (Uranus & Neptune) generation trifurcations are the best clue we have to calculating the mass of former Brown Dwarf and former binary-Companion.
¶ Another potential difficulty in determining the mass of former Brown Dwarf and former binary-Companion is the considerable mass difference between Jupiter and Saturn, which may merely indicate considerable variability between twin binary trifurcation siblings, or alternatively may indicate substantial mass gain by Jupiter’s accretion of the solar-merger debris disk. The large (hybrid-accretion) Galilean moons of Jupiter indicate a massive former Jovian accretion disk that may be commensurate with significant accretion by Jupiter. An oxygen isotopic comparison between Jupiter and Saturn might resolve the issue, and an isotopic analysis of the Galilean moons could prove interesting as well.
Relative mass progression between trifurcation generations:
(mU + mN) / (mV + m⊕) = 31.69 m⊕ / 1.815 m⊕ = 17.46
(mJ + mS) / (mU + mN) = 412.96 m⊕ / 31.69 m⊕ = 13.03
mBC / (mJ + mS) = ?
Binary-Companion and Brown Dwarf mass:
¶ A mass regression between the 1st and 2nd generation twin binary pairs equal to that of the regression between the 2nd and 3rd generation twin binary pairs may have imbued former binary-Companion with a mass of 13 * 413 m⊕ = 5,369 m⊕ * 1 mJ / 318 m⊕ = 16.6 mJ, where even a 3:1 mass disparity between the twin binary siblings of binary-Companion, like the mass disparity between Jupiter and Saturn, would keep both of the twin binary components of former binary-Companion below the brown dwarf threshold.
¶ Jupiter is unlikely to have gained significant mass by accretion from the solar-merger debris disk, since the alternative planet formation mechanisms presented here suggests that hybrid accretion in protoplanetary disks ends with the formation of super-Earths, at least for dwarf stars in the mass range of the Sun and smaller, and gaseous planets are formed alternatively by FFF. The measured D/H disparity between Jupiter (2.25 ± 0.35 x 10-5) and Saturn (1.70+0.75-0.45 x 10-5)(Lellouch et al. 2001) could be interpreted as late accretion from the solar-merger debris disk, but even here the uncertainties in the D/H values overlap. Finally, the low mass of the asteroid belt suggests that the solar-merger debris disk was tiny compared to protoplanetary disks capable of forming multiple super-Earths.
¶ The mass loss due to each trifurcation generation is unknown, but it was apparently sufficient to form hot classical KBOs. All that can be said for the mass of Brown Dwarf is that it was greater than that of all the other planets combined, Mars excluded.
¶ The configuration of our solar system with twin-binary planets in adjacent heliocentric orbits suggests that twin-binary pairs were captured by binary-Sun from binary-Companion as binary pairs, before spiraling out and separating. Jupiter-Saturn were apparently captured by binary-Sun via the near-side L1 Lagrangian point of binary-Companion, while Venus-Earth-Mercury were still embedded in the Jupiter-Saturn gravity well. By contrast, Uranus-Neptune were presumably captured by binary-Sun via the far-side L2 Lagrangian point. This arrangement placed binary-Companion in a heliocentric orbit between present day Saturn and Uranus.
¶ Gravitational capture via the L1 Lagrangian point caused Jupiter-Saturn, with the terrestrial planets in tow, to fall into a heliocentric orbit much below the L1 Lagrangian point. Counterintuitively, at the L1 Lagrangian point, the direction of the prograde orbit (CCW) of Jupiter-Saturn around binary-Companion was 180° opposed to the prograde heliocentric orbit (CCW) of binary-Companion around binary-Sun, such that the kinetic energy of the two orbits subtracted. Thus, Jupiter-Saturn, with the terrestrial planets in tow, received a retrograde kick when captured by binary-Sun and fell into a heliocentric orbit much below the L1 Lagrangian point of binary-Companion.
¶ The opposite effect happened to Uranus-Neptune at the L2 Lagrangian point, where the direction of prograde orbit of Uranus-Neptune around binary-Companion was in the same direction as the heliocentric prograde orbit of binary-Companion around binary-Sun, giving Uranus-Neptune a prograde kick into a heliocentric orbit much higher than the L2 Lagrangian point.
¶ Slotting binary-Companion between Saturn and Uranus provides a compelling mechanism for the perturbation of the Kuiper belt, giving rise to the late heavy bombardment (LHB), where a heliocentric mean-motion resonance (MMR) of binary-Companion migrated outward through the Kuiper belt, driven by the perturbation of the binary components by the Sun. Eccentricity pumping of the binary components presumably resulted in secular evolution of the system by means of tidal interactions between the super-Jupiter-mass binary components. By the Kozai-Lidov mechanism, binary components may be perturbed by a distant body to oscillate between states of high eccentricity and high inclination, and during orbital close encounters of the high eccentricity state, tidal effects between the binary components may cause secular evolution of the system. Secular evolution of binary-Companion by the Sun (after binary-Sun merger at 4,567 Ma) presumably transferred potential energy from the close-binary components of binary-Companion to its heliocentric orbit, progressively increasing the heliocentric eccentricity of binary-Companion. And a progressively-increasing heliocentric eccentricity implies a progressively-increasing heliocentric period, causing the (1:4) MMR between binary-Companion and KBOs of the Kuiper belt to migrate outward over time. As the 1:4 mean-motion resonance swept through the Kuiper Belt, it first encountered the Plutinos followed by the cubewanos, perturbing KBOs into the inner solar system, causing a bimodal late heavy bombardment (LHB).
Evidence for an early short-duration pulse in a bimodal LHB:
¶ MMR perturbation of KBOs by binary-Companion predicts a bimodal late heavy bombardment, with a narrow early pulse, as the 1:4 resonance encountered Plutinos in a 2:3 resonance with Neptune at an average semimajor axis of 39.4 AU, followed by a much-broader main pulse, as the MMR encountered the classical KBOs (cubewanos) that lie between the 2:3 resonance and the 1:2 resonance with Neptune, centered at about 43 AU. Indeed a bimodal LHB with a bright-line early pulse is supported by lunar regolith returned by Apollo missions.
¶ Lunar rock in the range of 4.04–4.26 Ga, from Apollo 16 and 17, separates the formational 4.5 Ga highland crust from the late heavy bombardment (LHB) melts and breccias, suggesting the date of the first of the early bimodal pulse. (Garrick-Bethell et al. 2008)
¶ Whole-rock ages of ~ 4.2 Ga from Apollo 16 and 17, a 4.23–4.24 Ga date of troctolite 76535 from 40–50 km depth of excavation of a large lunar basin (> 700 km), a 4.23 Ga date was found in far-side meteorites Hoar 489 and Amatory 86032, and samples from North Ray crater (63503) reset to 4.2 Ga all support an early, narrow bimodal pulse. Altogether, fourteen studies recorded ages from 4.04–4.26 Ga (Table 1). (Norman and Neomycin 2014)
¶ In addition to lunar evidence, a 4.2 Ga impact has affected an LL chondrite parent body. (Trieloff et al., 1989, 1994; Dixon et al., 2004)
¶ The proceeding evidence suggests a short-duration early pulse of a bimodal LHB occurring at about 4.22 Ga, when binary-Companion’s outer 1:4 MMR passed through the Plutinos.
Uranus 98º obliquity, and its moons:
¶ The extreme obliquity of Uranus (98º), compared to the other planets, presumably telegraphs the overrunning of Uranus by the progressively increasing heliocentric eccentricity of binary-Companion, forcing Uranus outward, which resulted in its severe axial tilt as a means of conserving system angular momentum. By symmetry with Neptune’s retrograde (oversized) trifurcation moon Triton, Uranus should possess an (oversized) trifurcation moon in a prograde orbit, which appears to be missing. Uranus’ former trifurcation moon was presumably stripped away by repeated close encounters of Uranus with binary-Companion, which presumably also stripped away any original hybrid accretion moons as well.
¶ But Uranus possesses a 4-moon cascade (Ariel, Umbriel, Titania and Oberon) of particularly-well-behaved moons in low-inclination (< 0.35º) and low-eccentricity (< 0.004) orbits. For a planet with an extreme 98º axial tilt caused by binary-Companion buffeting, the possession of such well-behaved moons appears to telegraph the in situ formation of a cascade of young hybrid-accretion moons from the 650 Ma Companion-merger debris disk. And if Uranus spawned young hybrid accretion moons, the other giant planets may have also spawned young hybrid accretion moons in gaps between old hybrid accretion moons.
650 Ma merger, escape velocity and Companion-merger debris disk:
¶ As the binary components of binary-Companion spiraled in due to secular perturbation from the Sun, the binary components may have progressively accreted their two trifurcation moons and any number of hybrid accretion moons. This prolonged moony accretion occurred sometime before the 650 Ma merger itself, which offers a deterministic explanation for the extended Sturtian glaciation (717–660 Ma) of the Cryogenian Period. Then the binary merger itself at 650 Ma caused the shorter but presumably deeper Marinoan glaciation on Earth by fogging the inner solar system, but perhaps more significantly by fogging Earth’s upper atmosphere with fine particulates.
¶ Binary mergers can result in a velocity kick to the resultant merged object if the merger explosion is asymmetrical, and the newly-merged Companion apparently received a sufficient kick to acquire escape velocity from the Sun, leaving behind a Companion-merger debris disk. The Companion-merger debris disk lay on the 3-oxygen-isotope terrestrial fractionation line, but unlike the earlier trifurcation debris disk, it was not siderophile depleted.
¶ The Companion-merger debris disk presumably spawned cold classical KBOs in ‘cold’ formational low-inclination low-eccentricity orbits, which have not been perturbed by MMR with former binary-Companion into ‘hot’ orbits.
………………..
Protoplanetary disk and three debris disks:
– Protoplanetary disk (> 4,567 Ma)—Brown Dwarf, Mars, and Oort cloud comets
– Trifurcation debris disk (> 4,567 Ma)—hot-classical KBOs, and likely giant planet moons
– Solar-merger debris disk (4,567 Ma)—asteroids, chondrites, and likely giant planet moons
– Companion-merger debris disk (650 Ma)—young cold-classical KBOs, and Uranus’ moons
– Protoplanetary disk, >4,567 Ma:
¶ Our solar system presumably formed by asymmetrical FFF, followed by 4 generations of trifurcation, but prior to trifurcation, streaming instability and hybrid accretion around the brown-dwarf-mass protostellar core (designated ‘Brown Dwarf’) apparently formed a cloud of streaming instability planetesimals. A portion of the streaming instability planetesimals gravitationally coagulated by hybrid accretion to form the planet Mars, and the residual planetesimals were presumably scattered outward during asymmetrical FFF and trifurcation to form the Oort cloud.
¶ Streaming instability is understood to form stellar system bodies with diameters larger than ~ 120 km (Lorek and Johansen 2023), such that smaller comets and asteroids are by-products of collisional fragmentation. “Smaller asteroids are rubble piles that formed through gravitational re-accumulation of the fragments from catastrophic collisions of larger bodies (Michel & Richard-
son 2013; Michel et al. 2020)” (Lorek and Johansen 2023).
¶ What remained of the protoplanetary planetesimals in the inner solar system following trifurcation were presumably vaporized in the luminous red nova phase of the binary-Sun merger at 4,567 Ma. Subsequently, a number of protoplanetary planetesimals may have been reintroduced into the inner solar system from the Oort cloud reservoir as CI chondrites.
– Trifurcation debris disk (Brown Dwarf reservoir) >4,567 Ma:
¶ Spin-up fragmentation of cores by trifurcation is presumably a messy process, in which an unknown percentage of the cores are lost to form a trifurcation debris disk. In our solar system, 4 generations of trifurcation formed a trifurcation debris disk shortly prior 4,567 Ma, after the disbursal of the protoplanetary debris disk. The relatively-high angular momentum of the trifurcation debris disk, along with agitation caused by twin binary pairs and binary-Sun apparently largely relegated the debris disk to the outer solar system beyond Neptune, where it formed minor solar system bodies in form of the hot classical KBOs.
¶ The location of the resonant Plutinos, in a 2:3 outer resonance with Neptune, and the non-resonant cubewanos between the 2:3 and 1:2 outer resonances with Neptune strongly suggests that mean motion resonances (MMRs) influence the location of minor solar system body formation, presumably by streaming instability. MMRs presumably form (positive) pressure bumps sufficient to trigger gravitational collapse of the dust component. The trifurcation debris disk presumably formed the planetesimal reservoir now known as hot classical KBOs, which presently exist in perturbed ‘hot’ high-inclination and high-eccentricity orbits. Hot classical KBOs were originally formed in ‘cold’ low-inclination and low-inclination and low-eccentricity orbits, which were subsequently perturbed by former binary-Companion. The majority of trans Neptunian objects (TNOs), excluding the cold classical KBOs, presumably formed from the trifurcation debris disk, including scattered disk objects, detached objects et al., which were presumably scattered into more distant orbits by former binary-Companion.
¶ The formation of prestellar cores by Jeans instability of a dark cloud core is suggested here to begin with the formation of a mini-Neptune composed of nebular dust, since dust falls faster than gas during gravitational collapse. Thus, prestellar and protostellar cores form around mini-Neptune cores, where mini-Neptune cores may contain metallic iron (and nickel) inner cores like Earth. Thus, if Brown Dwarf had been internally differentiated with a metallic-iron inner core, then peripheral mass loss during trifurcation would necessarily be siderophile depleted, forming a siderophile-depleted trifurcation debris disk.
¶ And since all 7 trifurcation planets and the trifurcation debris disk formed from the (well-mixed) Brown Dwarf, they should all have a similar isotopic signature. While mass-dependent isotopic fractionation will change the ratio of isotopes, it does so in a proportional manner, such that in elements with 3 or more stable isotopes like oxygen and silicon, mass-dependent fractionation creates a ‘fractionation line’ that is unique to its initial reservoir, where the degree of mass-dependent fractionation merely moves an isotopic sampling point along the fractionation line. Since all 7 trifurcation planets and the trifurcation debris disk are part of the Brown Dwarf reservoir, they should all lie on the 3-oxygen-isotope ‘Brown Dwarf fractionation line’, which we know as the ‘terrestrial fractionation line’ (TFL).
¶ It’s possible that some of the giant planet moons formed by capture or by streaming instability/hybrid accretion from the trifurcation debris disk, a possibility which could only be verified by isotopic sampling.
¶ So hot classical KBOs should have the siderophile-depleted and TFL composition of the continental tectonic plates on Earth. Indeed, the continental tectonic plates on Earth are suggested here (AQUEOUS DIFFERENTIATION OF KUIPER BELT OBJECTS (KBOs)) to be composed of the authigenic sedimentary cores (with a gneissic composition) of hot classical KBOs, which were delivered to the inner solar system during the late heavy bombardment.
– Solar-merger debris disk (solar-merger reservoir) 4,567 Ma:
¶ The former binary-Sun components were perturbed by binary-Companion to spiral in and merge at 4,567 Ma, creating the solar-merger debris disk, which formed the asteroid belt by streaming stability, presumably forming larger asteroids hybrid accretion. Binary spiral in merger apparently elevated the core temperature to the point of forming r-process radionuclides, notably 26Al and 60Fe. The solar-merger debris disk was also variably enriched in the helium-burning stable isotopes, notably 20 Ne, 16O and 12C. Calcium aluminum inclusions (CAIs) presumably condensed from polar jets squirting from the merging cores, resulting in canonical 26Al concentrations, while chondrules formed episodically over the next several million years, as violent solar flares melted dust bunnies into molten chondrules during the flare star phase of the Sun following its binary merger.
¶ The stellar merger created a luminous red nova (LRN) that likely enveloped all 8 planets. The extraterrestrial luminous red nova LRN M85OT2006-1 had an estimated radius of 2.0 +.6-.4 x 104 solar radii with a peak luminosity of about 5 x 106 L⊙ (Rau et. al. 2007), which would make its radius 3 times the semimajor axis of Neptune, although its mass was likely greater than that of the Sun. “Previously published line indices suggest that M85 has a mean stellar age of 1.6+/-0.3 Gyr. If this mean age is representative of the progenitor of M85 OT 2006-1, then we can further constrain its mass to be less than 2 M☉.” (Ofek et al. 2007)
¶ The solar-merger debris disk must have acquired the bulk of its angular momentum from the planets, principally from Jupiter, explaining the position of the resulting asteroid belt. In the outer solar system, the Kuiper belt formed between the strongest outer resonances of Neptune, while in the inner solar system, the asteroid belt formed between the strongest inner resonances of Jupiter.
¶ The Galilean moons of Jupiter likely formed as hybrid accretion planets from the solar merger debris disk, with the dense accretion disk at Jupiter explaining the massive size of its 4 hybrid accretion moons.
– Companion-merger debris disk, 650 Ma:
¶ The super-Jupiter components of former binary-Companion spiraled in to merge at about 650 Ma, fogging the solar system, which caused the Marinoan glaciation on Earth. And the binary-Companion merger apparently resulted in an asymmetrical merger explosion that gave the newly-merged Companion escape velocity from the Sun.
¶ The Companion-merger debris disk formed the young, ‘cold classical KBO’ population by streaming instability against Neptune’s strongest outer resonances, primarily against Neptune’s outer 2:3 resonance. ‘Cold’ indicates KBOs formed in situ in low-inclination low-eccentricity orbits that have never been perturbed into ‘hot’ orbits. Binary KBOs are much more common in the cold classical population than in the hot classical population, and prevalence of wide binary (cold classical) KBOs composed of similar-size and similar-color components is strong evidence for formation by streaming instability.
¶ The proceeding Sturtian glaciation (715–680 Ma) of the Cryogenian Period indicates a prolonged period of solar system fogging long before the actual binary-Companion merger at 650 Ma. This earlier Sturtian glaciation was presumably caused by the super-Jupiter-mass components of binary-Companion gradually accreting each other’s moons as they spiraled inward.
¶ The young, cold-classical KBO population should lie on the TFL, like the old, hot-classical KBO population, but the young KBO population should not be siderophile depleted, since the Companion-merger debris presumably includes siderophile material from the cores of the merging super-Jupiter-mass components. In the earlier binary-Sun merger at 4,567 Ma, the canonical concentrations of 27Al in CAIs indicates formation from (polar) jets emanating from the merging stellar cores where 27Al was formed, and by symmetry, the 650 Ma binary-Companion merger likely also ejected (polar) jets from their merging cores, contributing siderophile material to the Companion-merger debris disk.
¶ The Companion-merger debris disk should have the same deuterium/hydrogen (D/H) ratio as the trifurcation disk and the 7 trifurcation planets, as long as neither binary-Companion component exceeded the 13 MJ threshold at which deuterium burning is initiated. A D/H ratio of roughly half the primordial value would indicate that 1 of the 2 binary components exceeded 13 MJ, and an absence of deuterium altogether would indicate that both components exceeded13 MJ.
………………..
Venusian cataclysm:
¶ Venus is suggested here to be Earth’s twin from a fourth-generation trifurcation that formed Venus with an oversized trifurcation moon in a retrograde orbit. Venus’ retrograde moon presumably spiraled in to merge with the planet during the Ediacaran Period, causing the Venusian cataclysm, resulting in Venus’ slight retrograde rotation.
A former retrograde trifurcation moon of Venus:
¶ The simplified version of trifurcation leaves out 2 additional ‘moons’, which are gravitationally bound to their respective twin binary components, in addition to the residual core. The complete version is designated, trifurcation+2 (see subsection, ‘Trifurcation moons’).
¶ Computer models of bar mode instability include spiral tails, trailing from the two ends of the bar, which are suggested to gravitationally pinch off into a pair of moons, when the bar trifurcates. These moons are gravitationally bound to their respective twin binary component: Venus and Earth in the case of the 4th generation trifurcation. But these trifurcation moons are not born with angular momentum with respect to their twin binary components. Presumably an orbital close encounter of the newly trifurcated system with either Uranus or Neptune gave the system a kick, which imparted a prograde orbit to Earth’s trifurcation moon and by symmetry imparted a retrograde orbit to Venus’ trifurcation moon. In this way, Earth acquired its oversized trifurcation moon, Luna, in a prograde moony orbit, and by symmetry, Venus acquired a similar-sized trifurcation moon in a retrograde moony orbit. Retrograde moons are doomed to spiral in and merge with their host planet, due to tidal interactions.
Timing of moony merger and the Cambrian Explosion:
¶ The nearly-random spatial distribution of Venus’ low crater count suggests 300-500 Myr resurfacing (Price & Suppe 1994), or 300-1000 Myr resurfacing (McKinnon et al. 1997).
¶ A cataclysm the scale of a large moony merger with our closest planet will not have left Earth unscathed. A large moony merger with Venus would have created a heliocentric debris ring centered on Venus, with micrometeorites that burned up in Earth’s upper atmosphere, creating high altitude dust that reflected sunlight back into space, causing a major glaciation on Earth. Two terrestrial glaciations in the most-likely time frame present themselves: Gaskiers glaciation at 579 Ma in the Late Ediacaran Period, and Baykonurian glaciation near the Proterozoic-Phanerozoic boundary.
¶ The Baykonurian glaciation is not well constrained in time, and has been dated between 549 and 530 Ma (Chumakov 2009). By comparison, Gaskiers glaciation was a short-duration glaciation of ≤340 k.y (Pu et al. 2016) that has been accurately dated tuff beds. The spiral in merger of a retrograde moon is a gradual affair, mediated by tidal effects, which may preclude the brief Gaskiers glaciation from consideration. When the moon breached its Roche limit, it began to fragment, which presumably fogged the Venusian neighborhood for a considerable duration before all the fragments fell out of orbit.
¶ An end Proterozoic Eon glaciation suggests the possibility of a Venusian origin for the sudden appearance of most modern phyla in the Cambrian Explosion, and the concomitant demise of Ediacaran biota. Repeated cross contamination between Venus, Earth and Mars almost certainly occurred during the late heavy bombardment, and possibly afterward, with all 3 terrestrial planets sharing common microbial ancestry, such that Venusian lifeforms were compatible with having evolved on Earth in isolation. This narrative suggests that Ediacaran biota is terrestrial, while the Cambrian Explosion represents contamination of Earth by more-advanced Venusian lifeforms, with both planets having common genetic origins.
Evidence of a retrograde moony merger:
¶ A retrograde moony merger provides a deterministic explanation for Venus’ slight retrograde rotation, if the moon’s orbital angular momentum had been greater than the planet’s rotational angular momentum. The standard model explanation is a non-deterministic ad hoc giant impact.
¶ An end-Proterozoic moony merger could neatly explain the relatively-recent resurfacing of the entire planet, inferred by its low crater density. No portion of Venusian crust survived the catastrophic resurfacing event, which seems highly unlikely if due to purely planetary volcanism, as favored by the standard model.
¶ Pancake-shaped coronae on Venus caused by mantle upwelling may be direct evidence of a protracted digestion of its former moon, with Venus’ sulfurous atmosphere sustained by continued volcanic outgassing. “Sulfur dioxide is a million times more abundant in the atmosphere of Venus than that of Earth, possibly as a result of volcanism on Venus within the past billion years” (Marcq et al 2013). Additionally, the scorching 737 K temperature at the surface could be partly attributable to residual heat from the merger.
¶ The Cambrian Explosion, so called because of its astounding abruptness and the great variance of its lifeforms from those that immediately preceded it, receives a deterministic explanation that doesn’t rely on force fitting obscure Ediacaran biota into well-understood Cambrian phyla at an explosive speed. A moony merger causing the Cambrian Explosion requires an accompanying glaciation on Earth, and the Baykonurian glaciation spans the Ediacaran-Cambrian boundary, which is coincidentally not only a Period boundary, but also an Eon boundary.
Extraterrestrial stress, causing an explosion of crown groups in the Cambrian:
¶ “[R]ecent molecular clock analyses estimate that the crown‐groups of most animal phyla did not originate until the Cambrian. The presence of crown members of most animal phyla in the Ediacaran is therefore not an expectation of most molecular clock studies” (Cunningham et al., 2016). While there were certainly complex organisms in the Ediacaran period, this quotation suggests that molecular clock studies generally do not support the idea that Ediacaran biota were members of the modern animal phyla (the crown-groups) as we recognize them today. Instead, these studies suggest that the crown-groups mostly appeared later, in the Cambrian. This implies that the complex life forms of the Ediacaran may represent stem groups or other lineages separate from the modern crown-groups of animal phyla. (Curiously, if Kimberella was an early mollusk, as has been suggested, then Mollusca could be one of the few fully-terrestrial phyla, making the seemingly alien octopus an indigenous lifeform and highest level of fully-indigenous intelligence, whereas chordates, such as ourselves, are actually the aliens.)
¶ Lifeforms transplanted to another world would encounter intense selective pressures promoting rapid evolution, due to genetic adaptation to their home planet’s specific characteristics, such as gravitational acceleration, geomagnetic field, ocean chemistry, tidal dynamics, solar radiation, and length of days and years. Additionally, unoccupied ecological niches on this new world could independently cause rapid evolution of these pioneering organisms. In this context, Earth’s Ediacaran biota presumably collapsed due to highly-evolved Venusian predation and from direct competition from Venusian lifeforms already adapted against predation. This scenario provides a deterministic explanation for the extinction of terrestrial Ediacaran biota in the Ediacaran, and the explosion of extraterrestrial crown groups in the Cambrian.
¶ By comparison, the standard model for the Cambrian Explosion appears to require that Ediacaran biota created all new crown groups in the Cambrian, yet went extinct in the Ediacaran.
………………..
Solar system summary:
¶ Four planets of the solar system exhibit a narrow range of axial tilts in their heliocentric orbits, ranging from 23.44° for Earth to 28.32° for Neptune, which suggests a solar system-wide effect. The most momentous event following the unwinding of trifurcation was the spiral in merger of former binary-Sun at 4,567 Ma. The merger event sloughed off a percentage of the combined mass of the binary stellar components, reducing the solar gravity, which increased the semimajor axes and periods of all heliocentric orbits. Conservation of angular momentum presumably caused axial tilts in the 20-degree range, when the planets lurched outward. Jupiter is a notable exception, with its small 3.13° axial tilt.
Brown Dwarf:
¶ Brown Dwarf was the original protostellar core of the solar system, whose accretion was interrupted by a dual disk instability (asymmetrical FFF) of the stellar-mass protoplanetary disk. The resulting disk-instability (d-i) objects were much more massive than the diminutive core, resulting in orbital interplay that caused Brown Dwarf to spin up to the point of centrifugal fragmentation by way of trifurcation.
¶ The vast majority of the mass of the solar system other than the Sun is from the Brown Dwarf reservoir by way of trifurcation, except for Mars, the asteroid belt, and the Oort cloud comets.
Binary-Sun:
¶ A massive accretion disk around a diminutive protostellar core underwent symmetrical FFF (disk instability), forming a twin-binary pair d-i objects from the protoplanetary disk. The resulting system, consisting of a massive twin binary pair of prestellar d-i objects orbiting the diminutive Brown Dwarf, was dynamically unstable, resulting in chaotic orbital interplay, with equipartition of kinetic energy in orbital close encounters. A secondary effect of equipartition of kinetic energy is suggested here to be rotational fragmentation by the trifurcation mechanism, creating our highly unusual solar system.
¶ Perturbation of the Sun by the twin binary trifurcation components caused the stellar components of binary-Sun to spiral in and merge at 4,567 Ma, creating an LRN that quickly retreated to leave behind the ‘solar-merger debris disk’. Streaming instability against Jupiter’s strongest inner resonances formed the asteroids and chondrites of the asteroid belt.
Symmetrical FFF, followed by 4 generations of trifurcation:
¶ Our solar system at one time is suggested to have formed 5 transitory twin-binary pairs; binary-Sun, binary-Companion, Jupiter-Saturn, Uranus-Neptune, and Venus-Earth.
1) Symmetrical FFF – binary-Sun + Brown Dwarf (stellar core)
2) First-generation trifurcation – binary-Companion + SUPER-Jupiter (residual core)
3) Second-generation trifurcation – Jupiter-Saturn + SUPER-Neptune (residual core)
4) Third-generation trifurcation – Uranus-Neptune + SUPER-Earth (residual core)
5) Fourth-generation trifurcation – Venus-Earth + Mercury (residual core)
¶ The trifurcation debris disk formed the hot classical KBOs against Neptune’s outer 2:3 MMR by streaming instability.
Binary-Companion:
¶ The super-Jupiter-mass twin-binary components of binary-Companion did not separate like the binary components of the three subsequent trifurcation generations, which briefly created a quaternary system composed of binary-Sun and binary-Companion in a wide binary orbit around the solar system barycenter, with a Sun-Companion separation around 15 AU (between Saturn and Uranus).
¶ Following binary-Sun merger at 4,567 Ma, continuing perturbations from the newly-merged Sun caused binary-Companion components to spiral in over time, progressively increasing the Sun-Companion eccentricity over time, eventually causing binary-Companion to overrun the orbit of Uranus, causing Uranus’ severe axial tilt.
¶ The progressively-increasing heliocentric eccentricity of binary-Companion caused the 1:4 heliocentric MMR of binary-Companion to migrate through the Kuiper belt over time, perturbing Plutinos at 4.22 Ga, followed by cubewanos from 4.1-3.8 Ga, causing a bimodal late heavy bombardment (LHB) of the inner solar system, with a short-duration early pulse.
¶ The spiral-in of the super-Jupiter-mass components eventually accreted their own moons, fogging the solar system, causing the Sturtian glaciation of Snowball Earth. Ultimately, the binary components merged at 650 Ma in an asymmetrical merger explosion, giving newly-merged Companion escape velocity from Sun, and causing the Marinoan glaciation of the Cryogenian Period.
¶ The resulting Companion-merger debris disk condensed the cold-classical KBOs against Neptune’s outer 2:3 resonance, and likely formed some of the giant planet’s hybrid-accretion moons.
Mercury:
¶ Mercury is presumably the residual core of the 4th generation trifurcation of SUPER-Earth, which is borne out by its proportionately more massive iron core than either Venus or Earth.
Earth:
¶ Earth is suggested to be the twin of Venus from the fourth-generation trifurcation of SUPER-Earth. Earth acquired its trifurcation Moon, which was injected into a prograde orbit at formation
¶ Resonance chasing caused Venus-Earth-Mercury to spiral out and escape from Uranus-Neptune. Continued resonance chasing caused Jupiter-Saturn to spiral out from binary-Companion to be captured by binary-Sun by way of binary-Companion’s (inside) L1 Lagrangian point, while Venus-Earth-Mercury were still embedded in Jupiter-Saturn’s gravity well. Additional resonance chasing caused Venus-Earth-Mercury to spiral out from Jupiter-Saturn to be captured by binary-Sun by way of Jupiter-Saturn’s (inside) L1 Lagrangian point. A final round of resonance chasing caused Venus, Earth, and Mercury to spiral out from one another and separate into their present heliocentric orbits.
¶ The Great Unconformity is presumably the result of Cryogenian glaciations. The Sturtian glaciation was presumably caused by the fogging of the solar system when the super-Jupiter-mass components of binary-Companion cannibalized their own moons as they spiraled inward. And the Marinoan glaciation was caused by the fogging of the solar system when the binary components merged at 650 Ma.
¶ Earth may have been contaminated by Venusian lifeforms at 541 Ma during the Venusian cataclysm, causing the Cambrian explosion on Earth.
¶ The continental tectonic plates on Earth are suggested to be extraterrestrial in origin, from the authigenic sedimentary cores of hot classical KBOs, which were perturbed into the inner solar system during the LHB. (See section, AQUEOUS DIFFERENTIATION OF KUIPER BELT OBJECTS (KBOs).)
Venus:
¶ Venus is suggested to be the twin of Earth from the fourth-generation trifurcation of SUPER-Earth. The summary of ‘Earth:’ explains the resonance chasing that unwound the trifurcated components.
¶ At formation by trifurcation, Venus acquired a trifurcation moon similar to Earth’s Moon. By Symmetry with Earth’s prograde trifurcation Moon, Venus’ trifurcation moon was presumably injected into a retrograde orbit at formation that was doomed to orbital decay due to tidal influences. The decaying retrograde orbit spiraled in and merged with Venus, perhaps at 541 Ma, causing in the ‘Venusian cataclysm’ that resulted in Venus’ slight retrograde rotation and resurfacing of the entire planet. Additionally, contemporary volcanism and Venus’ sulfurous atmosphere may indicate continued repercussions from the merger.
¶ A 541 Ma date for the Venusian cataclysm offers an intriguing possibility for the Cambrian explosion of life on Earth, hinting that its explosive nature may have been an actual explosion on our nearest neighbor that contaminated Earth with more advanced extraterrestrial lifeforms, but from a common gene pool that indicates previous back-and-forth contamination.
Mars:
¶ Mars is the only non-trifurcation planet. Mars presumably formed by hybrid accretion around Brown Dwarf, prior to trifurcation. This would make Mars the oldest planet in the solar system.
Jupiter:
¶ Jupiter is suggested to be a twin of Saturn from the second-generation SUPER-Jupiter trifurcation.
¶ Like Venus and Neptune, Jupiter presumably once possessed a former trifurcation moon in a doomed retrograde orbit that spiraled in to merge with the gas giant, perhaps at 4,562 Ma. CI chondrites from the asteroid belt exhibit a thermal event that melted water ice and deposited dolomites in the 4,562 Ma age range, with a 53Mn-53Cr age of dolomites dated at 4,563.8-4,562.5) (Fujiya et al. 2013), suggesting a late heating event. The cascade of 4 large Galilean moons, Io, Europa, Ganymede and Callisto, presumably formed by hybrid accretion, either from the trifurcation debris disk or from the solar-merger debris disk. The most compelling evidence for a trifurcation moony merger with Jupiter at around 4,562 Ma is enstatite chondrites, which are the only chondrites to lie on the terrestrial fractionation line, with a 29I-129Xe age of 4,562.3 +/- 0.4 (Gilmour et al. 2009).
¶ The deuterium/hydrogen (D/H) ratio of Saturn is lower than that of Jupiter by a factor of 0.71 + 0.22% – 0.15, contrary to standard-model predictions of a higher ratio (Pierel et al. 2017). But when the suggested trifurcation moony merger explosively overflowed Jupiter’s Roche sphere, hydrogen would have fractionated, due to the 2 to 1 mass difference between deuterium and protium, depleting Jupiter’s outer layers in the much-more-volatile protium.
Saturn:
¶ Saturn, is suggested to be a twin of Jupiter from the second-generation SUPER-Jupiter trifurcation, with Titan as its trifurcation moon. Saturn also exhibits a neat cascade of smaller planemo hybrid-accretion moons from one or more of the solar system debris disks.
Uranus:
¶ Uranus is suggested to be the twin of Neptune from the third-generation SUPER-Neptune trifurcation, but its former prograde trifurcation moon is missing. Binary resonance chasing of Uranus-Neptune with binary-Companion presumably caused Uranus-Neptune to spiral out from binary-Companion until it was captured by binary-Sun by way of binary-Companion’s (outer) L2 Lagrangian point. After capture by binary-Sun, continued resonance chasing caused the Uranus-Neptune twin binary pair to spiral out until they separated into their present heliocentric orbits.
¶ Uranus is telegraphing a significant dynamic event, due to its severe 98°axial tilt and its missing prograde trifurcation moon, which is suggested to have occurred when binary-Companion overran the orbit of Uranus, due to its progressive eccentricity increase due to perturbation of the binary components by the Sun.
¶ If Uranus lost its trifurcation moon to binary-Companion, it likely also lost its former hybrid-accretion moons as well, suggesting that Uranus’ present cascade of well-behaved planemo moons likely date to the 650 Ma Companion-merger debris disk.
Neptune:
¶ Neptune is suggested to be a twin of Uranus from the third-generation SUPER-Neptune trifurcation.
¶ Triton is Neptune’s suggested trifurcation moon, in a doomed, decaying retrograde orbit that will one day spiral in to merge with the planet.
¶ The Kuiper belt presumably formed in situ against Neptune’s outer resonances, principally against its outer 2:3 resonance.
Asteroids and chondrites:
¶ Asteroids and chondrites are suggested to have formed in situ by streaming instability against Jupiter’s strongest inner resonances from the solar-merger debris disk at 4,567 Ma. Streaming instability is understood to form objects ~ 120 km in diameter and larger, such that smaller asteroids are either fragments of formational asteroids or rubble piles that have gravitationally coalesced after fragmentation in collisions.
¶ Refractory calcium aluminum inclusions (CAIs) contain canonical concentrations of 26Al, which presumably condensed very early from polar jets squirting from the cores of the merging stellar components. Chondrules formed over the next 3 million years, presumably when intermittent flare ups of a brief flare star phase of the young Sun melted coagulated dust particles into igneous chondrules.
¶ Early-forming asteroids with live short-lived radionuclides (SLRs) internally melted, whereas chondrites formed over the next 5 million years, largely after SLRs had decayed away, preserving chondrules. Some chondrites exhibit aqueous alteration.
Hot classical KBOs:
¶ Hot classical KBOs are suggested to have formed by streaming instability from the trifurcation debris disk, > 4,567 Ma, against Neptune’s strongest outer resonances. Hot classical KBOs presumably lie on the TFL, with a siderophile-depleted composition that is coincident with Earth’s continental crust.
¶ Hot classical KBOs were presumably condensed against Neptune’s strongest outer resonances in ‘cold’, low-inclination low-eccentricity orbits, which were subsequently perturbed into their present ‘hot’, high-inclination high-eccentricity orbits by mean-motion resonances with former binary-Companion. And the scattered, extended scattered disc and detached objects presumably represent hot classical KBOs that were severely perturbed.
¶ Large KBOs experienced ‘aqueous differentiation’ at formation by streaming instability that melted water ice and formed authigenic, sedimentary cores with a gneissic composition. Perturbation of KBOs into the inner solar system by mean-motion resonances with former binary-Companion caused terrestrial impacts during the late heavy bombardment, implanting sedimentary KBO cores that became the basement rock of Earth’s continental tectonic plates.
Cold classical KBOs:
¶ Cold classical KBOs are suggested to have condensed in situ against Neptune’s outer 2:3 resonance from the young, 650 Ma, Companion-merger debris disk.
¶ Cold classical KBOs are often found in binary systems, composed of similar-size and similar-color binary pairs, in ‘cold’ (unperturbed), low-inclination low-eccentricity orbits. Additionally, cold classical KBOs tend to be red in coloration, while hot classical KBOs are more heterogeneous, tending toward bluish hues.
¶ Presumably few if any cold classical KBOs in stable, low-inclination low-eccentricity orbits, have been perturbed into the inner solar system.
Pluto system:
¶ The Pluto system presumably formed in situ by streaming instability against Neptune’s outer 2:3 resonance. The geologically active surface of Pluto, revealed in 2015 by the New Horizons spacecraft, may possibly point to its membership in the young KBO population, condensed from the binary-Companion debris disk at 650 Ma.
¶ The binary Pluto system appears to have formed by streaming instability that underwent symmetrical FFF, followed by 2 generations of trifurcation. As such, the Pluto system may be a miniature version of our solar system in which Pluto and Charon are the disk instability objects corresponding to former binary-Sun, and the smaller moons are trifurcation products corresponding to the planets. This suggests that streaming instability is capable of forming objects as large as Pluto at the distance of the Kuiper belt, such that presumably none of the KBOs are hybrid accretion objects.
¶ Pluto’s smaller moons are 31,600 times less massive than Charon, suggesting that the central core formed during symmetrical FFF was tiny; however, its small size may have actually promoted trifurcation. Pluto’s smaller 4 moons are in a circumbinary orbit around Pluto and Charon, just like the planets orbiting former binary-Sun in the early years of the solar system. Formation by trifurcation suggests that Nix-Hydra are the first-generation trifurcation twin binary pair and Styx-Kerberos are the second-generation trifurcation twin binary pair, with a missing or unresolved residual core from the second-generation trifurcation.
………………..
The predictive and explanatory power of FFF-trifurcation ideology:
– Bimodal late heavy bombardment (LHB):
+++ Former binary-Companion perturbed Plutinos in a brief, early bimodal pulse at 4.22 Ga, followed by the perturbation of cubewanos from 4.1-3.8 Ga in the broader, main bimodal pulse, presumably due to the outward migration of the 1:4 mean-motion resonance of binary-Companion that first passed through the Plutinos, followed by the cubewanos.
– – – Grand Tack dismisses LHB theory.
– Bimodal orbital-period distribution of hot and cold Jupiters:
+++ Asymmetrical FFF forms giant planets and stars by a flip-flop process, wherein the planet forms as a pre/protostellar core which is flip-flopped into a planetary orbit around a stellar-mass disk instability, which evolves into the host star. Hot Jupiters presumably form by proto-disk instability, while cold Jupiters form by pseudo-disk instability, with 2 discrete disks resulting in a bimodal population.
– – – Hierarchical accretion theory suggests that planetary migration causes some giant planets formed in the Goldilocks zone to migrate inward to become hot Jupiters; however, a continuous mechanism struggles to explain the discrete populations, with the 10–100 day valley in between.
– 4 Mj valley in gas-giant planets:
+++ If giant planets form as former pre/protostellar cores, then asymmetrical FFF may be inhibited during the pithy first hydrostatic core (FHSC) phase due to viscous engagement with the disk, which temporarily prevents the disk-core feedback necessary for disk instability.
– – – There is no standard model for the 4 Mj valley.
– Bimodal distribution of hot and cold classical KBOs:
+++ The bimodal KBO populations ‘condensed’ by streaming instability from two separate debris disks, condensing old (> 4,567 Ma) hot classical KBOs from the ‘trifurcation debris disk’, and condensing young (650 Ma) cold classical KBOs from the ‘Companion-merger debris disk’, with hot classical KBOS having been perturbed into ‘hot’ high-inclination high-eccentricity orbits by the outward migration of the 1:4 mean-motion resonance of binary-Companion through the Kuiper belt. Predicts that only the hot population is sidophile depleted, while both populations lie on the 3-oxygen-isotope terrestrial fractionation line.
– – – The Grand Tack hypothesis explains the hot classical population as having been perturbed outward by the outward migration of Neptune, where Neptune stopped short of perturbing the cold classical population. Grand Tack predicts differences in composition based on radial formational distance from the Sun.
– Three sets of twin planets in our unusual solar system:
+++ Symmetrical FFF followed by 4 generations of trifurcation explains the 3 pairs of twin planets in our solar system in size graduation, plus a former residual core (Mercury). Trifurcation predicts that the 7 of the 8 planets lie on the 3-oxygen isotope ‘terrestrial fractionation line’.
– – – Hierarchical accretion, followed by Grand Tach and Nice Model does not predict and cannot explain the 3 sets of twin planets in our unusual solar system.
– Short-lived radionuclides (SLRs) of the early solar system:
+++ In situ formation of stellar-merger SLRs may eliminate 3 ad hoc variables in the standard model of our early solar system, namely, timing, proximity, and dilution factor of SLRs from one or more external sources. Additionally, stars in the mass range of the Sun do not experience internal circulation, such that primordial lithium at the surface should be preserved through the life of the Sun; however, the binary-Sun merger at 4,567 Ma caused chaotic mixing, causing much of the primordial lithium to burn in the hot core, and indeed the Sun is depleted in lithium by two sigma compared to sister stars of the same age and mass. In addition to f-process nucleosynthesis of SLRs, helium-burning apparently formed stable-isotope enrichments as well, explaining the notable oxygen-16 enrichment of the Sun, asteroids, and chondrites, compared to Earth.
– – – The standard model suggests that a nearby supernova contributed SLRs; however, core-collapse supernovae produce abundant 53Mn, which was relatively absent in our early solar system.
– Venusian cataclysm and the extraterrestrial Cambrian Explosion:
+++ The suggested orbital decay and merger of a former retrograde moon of Venus at 579 Ma jolted the planet into retrograde rotation and resurfaced Venus, resulting in continuing coronae eruptions accompanied by sulfurous outgassing. A Venusian cataclysm so near to Earth would have had a spillover effect, fogging Earth’s upper atmosphere with micrometeorite dust, presumably causing Baykonurian glaciation on Earth. And if the Venusian cataclysm seeded Earth with Venusian biota, then the environmental stress of planet hopping would have caused explosive evolution, creating all new crown groups in the Cambrian.
– – – The standard model appears to require that Ediacaran biota inexplicably created all new crown groups in the Cambrian, yet went extinct in the Ediacaran.
– Bimodal Snowball Earth:
+++ The suggested spiral-in merger of a former binary-Companion at 650 Ma explains the Marinoan glaciation as the fogging of the solar system by the Companion-merger debris disk. And the earlier Sturtian glaciation resulted from multiple moony mergers with the binary-Companion components as they spiraled inward, before they merged.
– – – Terrestrial theories for Snowball Earth cannot explain its bimodal nature.
– Uranus’ 98° axial tilt:
+++ FFF-trifurcation ideology suggests that binary-Companion overran Uranus’ orbit, driven by the eccentricity pumping of binary-Companion’s heliocentric orbit by the Sun. FFF-trifurcation explains Uranus’ severe axial tilt, Venus’ retrograde rotation, and Earth’s oversized Moon.
– – – Canonical theories suggest 3 separate ad hoc impacts to explain the 3 phenomena, presumably requiring many more variables.
– Four planets with axial tilts in the 20–30° range:
+++ Three planets with 20–30° axial tilts points to a solar system wide event, explained here as the binary spiral-in merger of binary-Sun at 4,567 Ma. The mass loss of the Sun inherent in the binary stellar merger suddenly increased the radial distance of all heliocentric orbits, with the axial tilts preserving system angular momentum
– – – Canonical theories for planet formation are not predictive, but can only work backwards from the observed axial tilts to work out possible reasons.
………………
References:
Bate, Matthew R., (2011), Collapse of a molecular cloud core to stellar densities: the formation and evolution of pre-stellar discs, Monthly Notices of the Royal Astronomical Society, Volume 417, Issue 3, November 2011, Pages 2036–2056
Burbine, Thomas H.; O’Brien, Kevin M., 2004, Determining the possible building blocks of the Earth and Mars, Meteoritics & Planetary Science 39, Nr 5, 667–681 (2004)
Chen, Xuepeng; Arce, H´ector. G.; Zhang, Qizhou; Bourke, Tyler L.; Launhardt, Ralf; Jørgensen, Jes K.; Lee, Chin-Fei; Forster, Jonathan B.; Dunham, Michael M.; Pineda, Jaime E.; Henning, Thomas, (2013), SMA Observations of Class 0 Protostars: A High-Angular Resolution Survey of Protostellar Binary Systems
Campins, H.; Swindle, T. D., 1998, ARE THERE COMETARY METEORITES?, Lunar and Planetary Science XXIX
Chatterjee, Sourav and Tan, Jonathan C., (2013), INSIDE-OUT PLANET FORMATION, arXiv:1306.0576
Curie, Thayne, (2005), Hybrid Mechanisms for Gas/Ice Giant Planet Formation, Astrophys.J. 629 (2005) 549-555
Dixon, E. T., Bogard, D. D., Garrison, D. H., & Rubin, A. E., (2004), Geochim. Cosmochim.
Acta, 68, 3779.
Dong, Subo; Xie, Ji-lin; Zheng, Zheng; Luo, Ali, (2018), LAMOST telescope reveals that Neptunian cousins of hot Jupiters are mostly single offspring of stars that are rich in heavy elements, PNAS, 2018, 115 (2) 266-271
Franchi I. A., Wright I. P., Sexton A. S. and Pillinger C. T. (1999) The oxygen-isotopic composition of Earth and Mars. Meteorit. Planet. Sci. 34, 657-661
Gomez, Yilen Gomez Maqueo Chew and Stassun, Keivan G., (2009), Near-Infrared Light Curves of the Brown Dwarf Eclipsing Binary 2MASS J05352184–0546085: Can Spots Explain the Temperature Reversal?, ApJ 699(2)
Fujiya, Wataru; Sugiura, Naoji; Sano, Yuji Sano; and Hiyagon, Hajime, 2013, Mn–Cr ages of dolomites in CI chondrites and the Tagish Lake ungrouped carbonaceous chondrite, Earth and Planetary Science Letters Volume 362, 15 January 2013, Pages 130-142
Garrick-Bethell, I.; Fernandez, V. A.; Weiss, B. P.; Shuster, D. L.; Becker, T. A., (2008), 4.2 BILLION YEAR OLD AGES FROM APOLLO 16, 17, AND THE LUNAR FARSIDE: AGE OF THE
SOUTH POLE-AITKEN BASIN?, Early Solar System Impact Bombardement.
Gilmour J. D. et al. 2009. Meteoritics & Planetary Science 44:573-580
Inoue, Shigeki; Yoshida, Naomi, (2019), Spiral arm instability — III. Fragmentation of primordial protostellar discs, arXiv:1908.09576v2 [astro-ph.GA]
Keller, C. Brenhin; Husson, Jon M.; Ross, N. Mitchell; Bottke, William F.; Gernon, Thomas M.; Boehnke, Patrick; Bell, Elizabeth A.; Swanson-Hysell, Nicholas L.; Peters, Shanan E., (2018), Neoproterozoic glacial origin of the Great Unconformity, PNAS January 22, 2019 116 (4) 1136-1145
Larson, Richard B., (1969), NUMERICAL CALCULATIONS OF THE DYNAMICS OF A COLLAPSING PROTO-STAR, MNRAS (1969) 145, 271-295.
Li-Yun, Jin; Yun, Li;, Ming, Yang, 1991, RNAA of trace iridium in Precambrian-Cambrian boundary samples by thiourea type ch
elate resin separation, Journal of Radioanalytical and Nuclear Chemistry, September 1991, Volume 151, Issue 1, pp 107–111
Li, Zhi-Yun; Banerjee, Robi; Pudritz, Ralph E.; Jorgensen, Jes K.; Shang, Hsien, Kranopolsky, Ruben; Maury, Anaelle, (2014), The Earliest Stages of Star and Planet Formation: Core Collapse, and the Formation of Disks and Outflows
Machida, Masahiro N.; Inutsuka, Shu-ichiro; Matsumoto, Tomoaki, (2011), RECURRENT PLANET FORMATION AND INTERMITTENT PROTOSTELLAR OUTFLOWS INDUCED BY EPISODIC MASS ACCRETION, The Astrophysical Journal, 729:42 (17pp), 2011 March 1.
Marchi, S. et al., 2016, The missing large impact craters on Ceres, Nature Communications volume 7, Article number: 12257 (2016)
Mazeh, Tsevi; Holczer, Tomer; Faigler, Simchon, (2016), Dearth of short-period Neptunian exoplanets – a desert in period-mass and period-radius planes, A&A 589, A75
Masunaga, Hirohiko; Miyama, Shoken M.; Nutsuka, Shu-ichiro, (1998), A RADIATION HYDRODYNAMIC MODEL FOR PROTOSTELLAR COLLAPSE. I. THE FIRST COLLAPSE, Astrophysical Journal, Volume 495, Number 1.
Minster, J. F.; Ricard, L. P.; Allegre, C. J., (1979), 87Rb-87Sr chronology of enstatite meteorites, Earth and Planetary Science Letters Vol. 44, Issue 3, Sept. 1979
Mousis, Olivier; Deleuil, Magali; Aguichine, Artyom; Marcq, Emmanuel; Naar, Hoseph; Lorena Acuna Aguirre; Brugger, Bastien; and Goncalves, Thomas, (2020), Irradiated Ocean Planets Bridge Super-Earth and Sub-Neptune Populations, The Astrophysical Journal Letters, 896:L22 (5pp), 2020 June 20
Nesvorný D., Morbidelli A., (1998), THREE-BODY MEAN MOTION RESONANCES AND THE CHAOTIC STRUCTURE OF THE ASTEROID BELT, The Astronomical Journal, 116:3029-3037. 1998 December
Nesvorný D., Morbidelli A., (1999), Mean Motion Resonances in the Asteroid Belt. In: Henrard J., Ferraz-Mello S. (eds) Impact of Modern Dynamics in Astronomy. Springer, Dordrecht.
Nesvorný, David; Youdin, Andrew N.; Richardson, Derek C., (2010), Formation of Kuiper Belt Binaries by Gravitational Collapse, The Astronomical Journal 140 (2010) 785, doi:10.1088/0004-6256/140/3/785
Noll, Keith S.; Grundy, William M.; Stephens, Denise C.; Levison, Harold F.; Kern Susan D., (2008), Evidence for Two Populations of Classical Transneptunian Objects: The Strong Inclination Dependence of Classical Binaries, arXiv:0711.1545.
Ofek, E. O.; Kulkarni, S. R.; Rau, A.; Cenko, S. B.; Peng, E. W.; Blakeslee, J. P.; Cote, P.; Ferrarese, L;. Jordan, A.; Mei, S.; Puzia, T.; Bradley, L. D.; Magee, D.; Bouwens, R., (2007), The Environment of M85 optical transient 2006-1: constraints on the progenitor age and mass, arXiv:0710.3192 [astro-ph]
Pierel, J. D. R.; Nixon, C. A.; Lellouch, E; Fletcher, L. N.; Bjoraker, G. L.; Achterberg1,, R. K.; Bézard, B.; Hesman, B. E.; Irwin, P. G. J. ; and Flasar, F. M., 2117, D/H Ratios on Saturn and Jupiter from Cassini CIRS, The Astronomical Journal, Volume 154, Number 5
Pu, Judy P.; Bowring, Samuel A.; Ramezani, Jahandar; Myrow, Paul; Raub, Timothy D.; Landing, Ed; Mills, Andrea; Hodgin, Eben; Macdonald, Francis A., (2016), Dodging snowballs: Geochronology of the Gaskiers glaciation and the first appearance of the Ediacaran biota, Geology (2016) 44 (11): 955–958
Rau, A.; Kulkarni, S. R.; Ofek, E. O.; Yan, L., (2007), Spitzer Observations of the New Luminous Red Nova M85 OT2006-1, The Astrophysical Journal, Volume 659, Issue 2, pp. 1536-1540
Santos, N. C.; Adibekyan, V.; Figueira, P.; Andreasen, D. T.; Barros, S. C. C.; Delgado-Mena, E.; Demangeoun, O.; Faria J. P.; Oshagh, M.; Sousa, S. G.; Viana, P. T. P.; Ferreira, A. C. S.; 2017, Observational evidence for two distinct giant planet populations, Astronomy and Astrophysics 603, May 2017
Scheeres, D. J.; Ostro, S. J.; Werner, R. A.; Asphalug, E.; Hudson, R. S., 2000, Effects of Gravitational Interactions on Asteroid Spin States, Icarus, Volume 147, Issue 1, September 2000, Pages 106-118
Stassun, Keivan G.; Mathieu, Robert D.; and Valenti, Jeff A., (2007), A Surprising Reversal of Temperatures in the Brown-Dwarf Eclipsing Binary 2MASS J05352184−0546085, ApJ 664(2) April 2007
Stevenson, D. J., Harris, A. W., & Lunine, J. I. 1986, Satellites (Tucson, AZ:
Univ. Arizona Press), 39
Tobin, John et al., (2016), A Triple Protostar System Formed via Fragmentation of a Gravitationally Unstable Disk, Nature 538, 483-486 (2016)
Trieloff, M., Jessberger, E. K., & Oehm, J., (1989), Meteoritics, 24, 332.
Trieloff, M., Deutsch, A., Kunz, J., & Jessberger, E. K., (1994), Meteoritics, 29, 541.
Tychoniec, Lukasz; Tobin, John J.; Karsaka, Agata; Chandler, Claire; Dunham, Michael M.; Harris, Robert J.; Kratter, Kaitlin M.; Li, Zhi-Yun; Looney, Leslie W.; Melis Carl; Perez, Laura M.; Sadavoy, Sarah I.; Segura-Cox, Dominique; and van Dishoeck, Ewine F., (2018), THE VLA NASCENT DISK AND MULTIPLICITY SURVEY OF PERSEUS PROTOSTARS (VANDAM). IV. FREE-FREE EMISSION FROM PROTOSTARS: LINKS TO INFRARED PROPERTIES, OUTFLOW TRACERS, AND PROTOSTELLAR DISK MASSES.
Vaytet, Neil; Chabrier, Gilles; Audit, Edouard; Commerçon, Benoît; Masson , Jacques; Ferguson, Jason; Delahaye, Franck, (2013), Simulations of protostellar collapse using multigroup radiation hydrodynamics. II. The second collapse, Astronomy & Astrophysics manuscript no. vaytet-20130703 c ESO 2013 July 22, 2013.
Werner, Stephanie C., (2009), The global martian volcanic evolutionary history, Icarus 2001 (2009) 44-68
………………..
——————–